Particle accelerator: Difference between revisions
No edit summary Tags: Mobile edit Mobile web edit |
|||
(957 intermediate revisions by more than 100 users not shown) | |||
Line 1: | Line 1: | ||
{{Short description|Research apparatus for particle physics}} |
|||
{{Redirect|Atom smasher}} |
|||
{{redirect-multi|2|Atom smasher|Supercollider}} |
|||
{{Pp-move-indef}} |
|||
[[Image:Fermilab.jpg|thumb|upright=1.5|The [[Tevatron|Tevatron (background circle)]], a [[synchrotron]] [[collider]] type particle accelerator at [[Fermi National Accelerator Laboratory]] (Fermilab), Batavia, Illinois, USA. Shut down in 2011, until 2007 it was the most powerful particle accelerator in the world, accelerating protons to an energy of over 1 [[TeV]] (tera electron volts). Beams of protons and antiprotons, circulating in opposite directions in the rear ring, collided at two magnetically induced intersection points. ]] |
|||
[[Image:2mv accelerator-MJC01.jpg|thumb|A 1960s single stage 2 MeV linear Van de Graaff accelerator, here opened for maintenance]] |
|||
[[File:Linear accelerator animation 16frames 1.6sec.gif|thumb|upright=2.2|Animation showing the operation of a [[linear particle accelerator|linear accelerator]], widely used in both physics research and cancer treatment.]] |
|||
A '''particle accelerator''' (or '''atom smasher''', in the early [[20th century]]<ref>{{cite web|url=http://science.howstuffworks.com/atom-smasher.htm||title=how stuff works{{ndash}} atom smasher}}</ref>) is a device that uses [[electric field]]s to propel [[ion]]s or [[electric charge|charged]] [[subatomic particle]]s to high speeds and to contain them in well-defined beams. An ordinary [[Cathode ray tube|CRT]] television set is a simple form of accelerator. There are two basic types: linear accelerators and circular accelerators. |
|||
A '''particle accelerator''' is a machine that uses [[electromagnetic field]]s to propel [[electric charge|charged]] [[particle]]s to very high speeds and energies to contain them in well-defined [[particle beam|beams]].<ref>{{cite book |last1=Chao |first1=Alexander W |url=http://www.worldscientific.com/worldscibooks/10.1142/7037 |title=Reviews of Accelerator Science and Technology: Volume 1 |last2=Chou |first2=Weiren |publisher=World Scientific |year=2008 |isbn=978-981-283-520-8 |location=Singapore |language=en |doi=10.1142/7037|bibcode=2008rast.book.....C }}</ref><ref> |
|||
This page describes types of particle accelerators. For a list of existing and historic particle accelerators see: |
|||
{{cite book |
|||
''[[List of accelerators in particle physics]]''. |
|||
|last1=Livingston |first1=M. S. | author-link1 = Milton Stanley Livingston |
|||
{{TOClimit|limit=3}} |
|||
|last2=Blewett |first2=J. |
|||
|year=1969 |
|||
|title=Particle Accelerators |
|||
|publisher=[[McGraw-Hill]] |
|||
|location=New York |
|||
|isbn=978-1-114-44384-6 |
|||
}}</ref> Small accelerators are used for fundamental research in [[particle physics]]. Accelerators are also used as [[synchrotron light source]]s for the study of [[condensed matter physics]]. Smaller particle accelerators are used in a wide variety of applications, including [[particle therapy]] for [[oncology|oncological]] purposes, [[Isotopes in medicine|radioisotope]] production for medical diagnostics, [[Ion implantation|ion implanters]] for the manufacturing of [[Semiconductor|semiconductors]], and [[Accelerator mass spectrometry|accelerator mass spectrometers]] for measurements of rare isotopes such as [[radiocarbon]]. |
|||
Large accelerators include the [[Relativistic Heavy Ion Collider]] at [[Brookhaven National Laboratory]] in New York, and the largest accelerator, the [[Large Hadron Collider]] near Geneva, Switzerland, operated by [[European Organization for Nuclear Research|CERN]]. It is a [[collider]] accelerator, which can accelerate two beams of protons to an energy of 6.5 [[TeV]] and cause them to collide head-on, creating center-of-mass energies of 13 TeV. There are more than 30,000 accelerators in operation around the world.<ref>{{Cite web |date=2016-10-12 |title=More background on accelerators |url=https://www.iaea.org/topics/nuclear-science/nuclear-research/accelerators/background |access-date=2023-11-10 |website=www.iaea.org |language=en}}</ref><ref>{{cite web|last=Witman|first=Sarah|title=Ten things you might not know about particle accelerators|url=http://www.symmetrymagazine.org/article/april-2014/ten-things-you-might-not-know-about-particle-accelerators|work=Symmetry Magazine|date=15 April 2014 |publisher=[[Fermi National Accelerator Laboratory]]|access-date=21 April 2014}}</ref> |
|||
==Uses== |
|||
[[Image:Particle accelerator DSC09089.JPG|thumb|[[Beamline]]s leading from the Van de Graaf accelerator to various experiments, in the basement of the [[Jussieu Campus]] in [[Paris]]]] |
|||
[[File:Cockcroft–Walton generator.jpg|thumb|In early particle accelerators a [[Cockcroft-Walton generator|Cockcroft-Walton voltage multiplier]] was responsible for voltage multiplying. This piece of the accelerator helped in the development of the [[Nuclear weapon|atomic bomb]]. Built in 1937 by [[Philips]] of [[Eindhoven]] it currently resides in the [[Science Museum (London)|National Science Museum]] in [[London]], [[England]].]] |
|||
[[Image:Weizmann Institute particle accelerator.jpg|thumb|The now disused Koffler particle accelerator at the [[Weitzmann Institute]], [[Rehovot]], [[Israel]]]] |
|||
Beams of high-energy particles are useful for both fundamental and applied research in the sciences. |
|||
For the most basic inquiries into the dynamics and structure of matter, space, and time, physicists seek the simplest kinds of interactions at the highest possible energies. |
|||
These typically entail particle energies of many [[GeV]], and the interactions of the simplest kinds of particles: [[lepton]]s (e.g. electrons and [[positron]]s) and [[quark]]s for the matter, or [[photon]]s and [[gluon]]s for the [[quantum field theory|field quanta]]. |
|||
Since isolated quarks are experimentally unavailable due to [[color confinement]], the simplest available experiments involve the interactions of, first, leptons with each other, and second, of leptons with [[nucleon]]s, which are composed of quarks and gluons. |
|||
To study the collisions of quarks with each other, scientists resort to collisions of nucleons, which at high energy may be usefully considered as [[Parton (particle physics)|essentially 2-body interactions]] of the quarks and gluons of which they are composed. |
|||
Thus elementary particle physicists tend to use machines creating beams of electrons, positrons, protons, and [[anti-proton]]s, interacting with each other or with the simplest nuclei (eg, hydrogen or [[deuterium]]) at the highest possible energies, generally hundreds of GeV or more. |
|||
[[Nuclear physicist]]s and [[cosmologist]]s may use beams of bare [[atomic nucleus|atomic nuclei]], stripped of electrons, to investigate the structure, interactions, and properties of the nuclei themselves, and of [[condensed matter]] at extremely high temperatures and densities, such as might have occurred in the first moments of the [[Big Bang]]. |
|||
These investigations often involve collisions of heavy nuclei{{ndash}} of atoms like iron or gold{{ndash}} at energies of several GeV per [[nucleon]]. |
|||
At lower energies, beams of accelerated nuclei are also used in medicine, as for the treatment of cancer. |
|||
There are two basic classes of accelerators: electrostatic and electrodynamic (or electromagnetic) accelerators.<ref> |
|||
Besides being of fundamental interest, high energy electrons may be coaxed into emitting extremely bright and coherent beams of high energy photons{{ndash}} ultraviolet and X ray{{ndash}} via [[synchrotron radiation]], which photons have numerous uses in the study of atomic structure, chemistry, condensed matter physics, biology, and technology. Examples include the [[ESRF]] in Europe, which has recently been used to extract detailed 3-dimensional images of insects trapped in amber.<ref>{{cite web|url=http://news.bbc.co.uk/2/hi/science/nature/7324564.stm |title=Secret 'dino bugs' revealed|publisher=BBC|author=Jonathan Amos|accessdate=2008-09-11}}</ref> |
|||
{{cite book |
|||
Thus there is a great demand for electron accelerators of moderate (GeV) energy and high intensity. |
|||
|last=Humphries |
|||
|first=Stanley |
|||
|year=1986 |
|||
|url=https://archive.org/details/principlescharge00hump/page/4 |
|||
|title=Principles of Charged Particle Acceleration |
|||
|page=[https://archive.org/details/principlescharge00hump/page/4 4] |
|||
|publisher=[[Wiley-Interscience]] |
|||
|isbn=978-0471878780 |
|||
}}</ref> ''[[Electrostatic particle accelerator]]s'' use static [[electric field]]s to accelerate particles. The most common types are the [[Cockcroft–Walton generator]] and the [[Van de Graaff generator]]. A small-scale example of this class is the [[cathode-ray tube]] in an ordinary old television set. The achievable [[kinetic energy]] for particles in these devices is determined by the accelerating [[voltage]], which is limited by [[electrical breakdown]]. ''Electrodynamic'' or ''electromagnetic'' accelerators, on the other hand, use changing electromagnetic fields (either [[Electromagnetic induction|magnetic induction]] or oscillating [[radio frequency]] fields) to accelerate particles. Since in these types the particles can pass through the same accelerating field multiple times, the output energy is not limited by the strength of the accelerating field. This class, which was first developed in the 1920s, is the basis for most modern large-scale accelerators. |
|||
[[Rolf Widerøe]], [[Gustav Ising]], [[Leó Szilárd]], [[Max Steenbeck]], and [[Ernest Lawrence]] are considered pioneers of this field, having conceived and built the first operational [[linear particle accelerator]],<ref>{{Cite book |last1=Sessler |first1=Andrew |url=http://www.worldscientific.com/worldscibooks/10.1142/8552 |title=Engines of Discovery: A Century of Particle Accelerators Revised and Expanded Edition |last2=Wilson |first2=Edmund |publisher=World Scientific |year=2014 |isbn=978-981-4417-18-1 |language=en |doi=10.1142/8552|bibcode=2014edcp.book.....S }}</ref> the [[betatron]], as well as the [[cyclotron]]. Because the target of the particle beams of early accelerators was usually the atoms of a piece of matter, with the goal being to create collisions with their nuclei in order to investigate nuclear structure, accelerators were commonly referred to as '''atom smashers''' in the 20th century.<ref> |
|||
===Low-energy machines=== |
|||
{{cite journal |
|||
Everyday examples of particle accelerators are [[cathode ray tube]]s found in television sets and [[X-ray]] generators. These low-energy accelerators generators use a single pair of [[electrode]]s with a [[direct current|DC]] voltage of a few thousand volts between them. In an X-ray generator, the target itself is one of the electrodes. |
|||
|date=April 1935 |
|||
A low-energy particle accelerator called an [[ion implanter]] is used in the manufacture of [[integrated circuit]]s. |
|||
|title=six Million Volt Atom Smasher Creates New Elements |
|||
|url=https://books.google.com/books?id=lNsDAAAAMBAJ&q=%22Six+Million+Volt+Atom+Smasher+Creates+New+Elements%22&pg=PA580 |
|||
|journal=[[Popular Mechanics]] |
|||
|page=580 |
|||
}}</ref> The term persists despite the fact that many modern accelerators create collisions between two [[subatomic particles]], rather than a particle and an atomic nucleus.<ref> |
|||
{{cite news |
|||
|last=Higgins |first=A. G. |
|||
|title=Atom Smasher Preparing 2010 New Science Restart |
|||
|url=https://www.usnews.com/science/articles/2009/12/18/atom-smasher-preparing-2010-new-science-restart.html |
|||
|date=December 18, 2009 |
|||
|publisher=[[U.S. News & World Report]] |
|||
}}</ref><ref> |
|||
{{cite journal |
|||
|last=Cho |first=A. |
|||
|date=June 2, 2006 |
|||
|title=Aging Atom Smasher Runs All Out in Race for Most Coveted Particle |
|||
|journal=[[Science (journal)|Science]] |
|||
|volume=312 |issue=5778 |pages=1302–1303 |
|||
|doi=10.1126/science.312.5778.1302 |
|||
|pmid=16741091 |
|||
|s2cid=7016336 |
|||
}}</ref><ref> |
|||
{{cite book |
|||
|year=2005 |
|||
|chapter=Atom smasher |
|||
|chapter-url=https://books.google.com/books?id=yKUagx8PB_EC&q=%22atom+smasher%22&pg=PA49 |
|||
|title=American Heritage Science Dictionary |
|||
|page=[https://archive.org/details/isbn_9780618455041/page/49 49] |
|||
|publisher=[[Houghton Mifflin Harcourt]] |
|||
|isbn=978-0-618-45504-1 |
|||
|title-link=American Heritage Science Dictionary |
|||
}}</ref> |
|||
== Uses == |
|||
===High-energy machines=== |
|||
[[Image:Particle accelerator DSC09089.JPG|thumb|[[Beamline]]s leading from the [[Van de Graaff accelerator]] to various experiments, in the basement of the [[Jussieu Campus]] in [[Paris]].]] |
|||
DC accelerator types capable of accelerating particles to speeds sufficient to cause nuclear reactions are [[Cockcroft-Walton generator]]s or [[voltage multiplier]]s, which convert AC to high voltage DC, or [[Van de Graaff generator]]s that use static electricity carried by belts. |
|||
[[File:SLAC National Accelerator Laboratory Aerial 2.png|thumb|Building covering the 2 mile (3.2 km) beam tube of the [[Stanford Linear Accelerator]] (SLAC) at Menlo Park, California, the second most powerful linac in the world.]] |
|||
Beams of high-energy particles are useful for fundamental and applied research in the sciences and also in many technical and industrial fields unrelated to fundamental research.<ref>{{Cite book|last=Möller|first=Sören|url=http://link.springer.com/10.1007/978-3-030-62308-1|title=Accelerator Technology: Applications in Science, Medicine, and Industry|date=2020|publisher=Springer International Publishing|isbn=978-3-030-62307-4|series=Particle Acceleration and Detection|location=Cham|language=en|doi=10.1007/978-3-030-62308-1|s2cid=229610872}}</ref> There are approximately 30,000 accelerators worldwide; of these, only about 1% are research machines with energies above 1 [[GeV]], while about 44% are for [[Radiation therapy|radiotherapy]], 41% for [[ion implantation]], 9% for industrial processing and research, and 4% for biomedical and other low-energy research.<ref> |
|||
The largest and most powerful particle accelerators, such as the [[RHIC]], the [[Large Hadron Collider]] (LHC) at CERN (which came on-line in mid-November 2009<ref name="CERN September"> |
|||
{{cite |
{{cite journal |
||
|last=Feder |first=T. |
|||
|author= |
|||
|year=2010 |
|||
|url=http://press.web.cern.ch/press/PressReleases/Releases2009/PR02.09E.html |
|||
|title=Accelerator school travels university circuit |
|||
|title=CERN management confirms new LHC restart schedule |
|||
|url=http://controls.als.lbl.gov/als_physics/Fernando/FSannibaleWebSite/Teaching/USPAS/USPASHighLights/PhysiscsTodayUSPAS_Feb2010.pdf |
|||
|date=9 February 2009 |
|||
|journal=[[Physics Today]] |
|||
|publisher=[[CERN]] Press Office |
|||
|volume=63 |issue=2 |pages=20–22 |
|||
|accessdate=2009-02-10 |
|||
|bibcode=2010PhT....63b..20F |
|||
}}</ref><ref name="CERN October"> |
|||
|doi=10.1063/1.3326981 |
|||
{{cite web |
|||
}}</ref> |
|||
|author= |
|||
|url=http://press.web.cern.ch/press/PressReleases/Releases2009/PR09.09E.html |
|||
=== Particle physics === |
|||
|title=CERN reports on progress towards LHC restart |
|||
|date=19 June 2009 |
|||
For the most basic inquiries into the dynamics and structure of matter, space, and time, physicists seek the simplest kinds of interactions at the highest possible energies. These typically entail particle energies of many [[GeV]], and interactions of the simplest kinds of particles: [[lepton]]s (e.g. electrons and [[positron]]s) and [[quark]]s for the matter, or [[photon]]s and [[gluon]]s for the [[quantum field theory|field quanta]]. Since isolated quarks are experimentally unavailable due to [[color confinement]], the simplest available experiments involve the interactions of, first, leptons with each other, and second, of leptons with [[nucleon]]s, which are composed of quarks and gluons. To study the collisions of quarks with each other, scientists resort to collisions of nucleons, which at high energy may be usefully considered as [[Two-body problem|essentially 2-body interactions]] of the quarks and gluons of which they are composed. This elementary particle physicists tend to use machines creating beams of electrons, positrons, protons, and [[antiproton]]s, interacting with each other or with the simplest nuclei (e.g., [[hydrogen]] or [[deuterium]]) at the highest possible energies, generally hundreds of GeV or more. |
|||
|publisher=[[CERN]] Press Office |
|||
|accessdate=2009-07-21 |
|||
The largest and highest-energy particle accelerator used for elementary [[particle physics]] is the [[Large Hadron Collider]] (LHC) at [[CERN]], operating since 2009.<ref name="CERNNovember"> |
|||
}}</ref><ref name="CERN November"> |
|||
{{cite |
{{cite press release |
||
|date=November 23, 2009 |
|||
|author= |
|||
|url=http://press.web.cern.ch/press/PressReleases/Releases2009/PR17.09E.html |
|||
|title=Two circulating beams bring first collisions in the LHC |
|title=Two circulating beams bring first collisions in the LHC |
||
|url=http://press.cern/press-releases/2009/11/two-circulating-beams-bring-first-collisions-lhc |
|||
|date=23 November 2009 |
|||
|publisher=[[CERN]] Press Office |
|publisher=[[CERN]] Press Office |
||
| |
|access-date=2009-11-23 |
||
}}</ref> |
|||
}}</ref>) and the [[Tevatron]], are used for experimental [[particle physics]]. |
|||
=== Nuclear physics and isotope production === |
|||
Particle accelerators can also produce proton beams, which can produce "proton-heavy" medical or research [[isotope]]s as opposed to the "neutron-heavy" ones made in fission reactors. An example of this type of machine is LANSCE at [[Los Alamos National Laboratory|Los Alamos]]. |
|||
[[Nuclear physicist]]s and [[cosmologist]]s may use beams of bare [[atomic nucleus|atomic nuclei]], stripped of electrons, to investigate the structure, interactions, and properties of the nuclei themselves, and of [[condensed matter]] at extremely high temperatures and densities, such as might have occurred in the first moments of the [[Big Bang]]. These investigations often involve collisions of heavy nuclei{{spaced ndash}}of atoms like [[iron]] or [[gold]]{{spaced ndash}}at energies of several GeV per [[nucleon]]. The largest such particle accelerator is the [[Relativistic Heavy Ion Collider]] (RHIC) at [[Brookhaven National Laboratory]]. |
|||
==Linear particle accelerators== |
|||
{{main|Linear particle accelerator}} |
|||
In a linear accelerator (linac), particles are accelerated in a straight line with a target of interest at one end. Linacs are very widely used{{ndash}} every [[cathode ray tube]] contains one. They are also used to provide an initial low-energy kick to particles before they are injected into circular accelerators. The longest linac in the world is the [[Stanford Linear Accelerator Center|Stanford Linear Accelerator]], SLAC, which is 3 km (2 miles) long. SLAC is an electron-[[positron]] collider. |
|||
Particle accelerators can also produce proton beams, which can produce proton-rich medical or research [[isotope]]s as opposed to the neutron-rich ones made in [[fission reactor]]s; however, recent work has shown how to make <sup>99</sup>[[Molybdenum|Mo]], usually made in reactors, by accelerating isotopes of hydrogen,<ref> |
|||
Linear high-energy accelerators use a linear array of plates (or drift tubes) to which an alternating high-energy field is applied. As the particles approach a plate they are accelerated towards it by an opposite polarity charge applied to the plate. As they pass through a hole in the plate, the polarity is switched so that the plate now repels them and they are now accelerated by it towards the next plate. Normally a stream of "bunches" of particles are accelerated, so a carefully controlled AC voltage is applied to each plate to continuously repeat this process for each bunch. |
|||
{{cite journal |
|||
| last1=Nagai | first1=Y. |
|||
| last2=Hatsukawa |first2=Y. |
|||
| year=2009 |
|||
| title= Production of <sup>99</sup>Mo for Nuclear Medicine by <sup>100</sup>Mo(''n'',2''n'')<sup>99</sup>Mo |
|||
| journal=[[Journal of the Physical Society of Japan]] |
|||
| volume=78 | issue=3 | pages=033201 |
|||
| doi = 10.1143/JPSJ.78.033201 | doi-access=free |
|||
| bibcode = 2009JPSJ...78c3201N |
|||
}}</ref> although this method still requires a reactor to produce [[tritium]]. An example of this type of machine is [[LANSCE]] at [[Los Alamos National Laboratory]]. |
|||
=== Synchrotron radiation === |
|||
As the particles approach the speed of light the switching rate of the electric fields becomes so high that they operate at microwave frequencies, and so [[Cavity resonator|RF cavity resonators]] are used in higher energy machines instead of simple plates. |
|||
[[Electron]]s propagating through a magnetic field emit very bright and coherent [[photon]] beams via [[synchrotron radiation]]. It has numerous uses in the study of atomic structure, chemistry, condensed matter physics, biology, and technology. A large number of [[synchrotron light source]]s exist worldwide. Examples in the U.S. are [[Stanford Synchrotron Radiation Lightsource|SSRL]] at [[SLAC National Accelerator Laboratory]], [[Advanced Photon Source|APS]] at Argonne National Laboratory, [[Advanced Light Source|ALS]] at [[Lawrence Berkeley National Laboratory]], and [[National Synchrotron Light Source II|NSLS-II]] at [[Brookhaven National Laboratory]]. In Europe, there are [[MAX IV Laboratory|MAX IV]] in Lund, Sweden, [[BESSY]] in Berlin, Germany, [[Diamond Light Source|Diamond]] in Oxfordshire, UK, [[European Synchrotron Radiation Facility|ESRF]] in [[Grenoble]], France, the latter has been used to extract detailed 3-dimensional images of insects trapped in amber.<ref> |
|||
Linear accelerators are also widely used in medicine, for [[radiotherapy]] and [[radiosurgery]]. Medical grade LINACs accelerate electrons using a [[klystron]] and a complex bending magnet arrangement which produces a beam of 6-30 million electron-volt ([[MeV]]) energy. The electrons can be used directly or they can be collided with a target to produce a beam of [[X-rays]]. The reliability, flexibility and accuracy of the radiation beam produced has largely supplanted the older use of [[Cobalt-60]] therapy as a treatment tool. |
|||
{{cite news |
|||
|last=Amos |first=J. |
|||
|date=April 1, 2008 |
|||
|title=Secret 'dino bugs' revealed |
|||
|url=http://news.bbc.co.uk/2/hi/science/nature/7324564.stm |
|||
|publisher=[[BBC News]] |
|||
|access-date=2008-09-11 |
|||
}}</ref> |
|||
[[Free-electron laser]]s (FELs) are a special class of light sources based on [[synchrotron radiation]] that provides shorter pulses with higher temporal [[Coherence (physics)|coherence]]. A specially designed FEL is the most [[Synchrotron light source#Brilliance|brilliant]] source of [[x-ray]]s in the observable universe.<ref>{{Cite journal|last1=Ullrich|first1=Joachim|last2=Rudenko|first2=Artem|last3=Moshammer|first3=Robert|date=2012-04-04|title=Free-Electron Lasers: New Avenues in Molecular Physics and Photochemistry|url=https://www.annualreviews.org/doi/10.1146/annurev-physchem-032511-143720|journal=Annual Review of Physical Chemistry|volume=63|issue=1|pages=635–660|doi=10.1146/annurev-physchem-032511-143720|pmid=22404584|bibcode=2012ARPC...63..635U|issn=0066-426X}}</ref> The most prominent examples are the [[SLAC National Accelerator Laboratory#LCLS|LCLS]] in the U.S. and [[European XFEL]] in Germany. More attention is being drawn towards [[X-ray#Soft and hard X-rays|soft x-ray]] lasers, which together with pulse shortening opens up new methods for [[Attophysics|attosecond science]].<ref>{{cite journal |last1=Mak |first1=Alan |last2=Shamuilov |first2=Georgii |last3=Salén |first3=Peter |last4=Dunning |first4=David |last5=Hebling |first5=János |last6=Kida |first6=Yuichiro |last7=Kinjo |first7=Ryota |last8=McNeil |first8=Brian W J |last9=Tanaka |first9=Takashi |last10=Thompson |first10=Neil |last11=Tibai |first11=Zoltán |date=2019-02-01 |title=Attosecond single-cycle undulator light: a review |url=https://iopscience.iop.org/article/10.1088/1361-6633/aafa35 |journal=Reports on Progress in Physics |volume=82 |issue=2 |pages=025901 |doi=10.1088/1361-6633/aafa35 |pmid=30572315 |bibcode=2019RPPh...82b5901M |s2cid=58632996 |issn=0034-4885 }}</ref> Apart from x-rays, FELs are used to emit [[Terahertz radiation|terahertz light]], e.g. FELIX in Nijmegen, Netherlands, TELBE in Dresden, Germany and NovoFEL in Novosibirsk, Russia. |
|||
====Accelerator pioneers==== |
|||
*[[John Cockcroft]] worked on a linear accelerator |
|||
*[[Robert J. Van de Graaff]] at [[Princeton University]] initially used Tesla coils and then in 1929 migrated to Van De Graff generators.<ref>[http://chem.ch.huji.ac.il/~eugeniik/history/graaff.html Van de Graaff biography]</ref> |
|||
Thus there is a great demand for electron accelerators of moderate ([[Electronvolt|GeV]]) energy, high intensity and high beam quality to drive light sources. |
|||
===Tandem electrostatic accelerators=== |
|||
In a tandem accelerator, the negatively charged ion gains power from the eartyh and all its beloning energy by attraction to the very high positive voltage at the geometric centre of the pressure vessel. When it arrives at the centre region known as the high voltage terminal, some electrons are stripped from the ion. The ion then becomes positive and accelerated away by the high positive voltage. Thus, this type of accelerator is called a 'tandem' accelerator. The accelerator has two stages of acceleration, first pulling and then pushing the charged particles. An example of a tandem accelerator is [[ANTARES (accelerator)|ANTARES]] (Australian National Tandem Accelerator for Applied ).ghc |
|||
=== Low-energy machines and particle therapy === |
|||
==Circular or cyclic accelerators== |
|||
Everyday examples of particle accelerators are [[cathode ray tube]]s found in television sets and [[X-ray]] generators. These low-energy accelerators use a single pair of [[electrode]]s with a [[direct current|DC]] voltage of a few thousand volts between them. In an X-ray generator, the target itself is one of the electrodes. A low-energy particle accelerator called an [[ion implanter]] is used in the manufacture of [[integrated circuit]]s. |
|||
In the circular accelerator, particles move in a circle until they reach sufficient energy. The particle track is typically bent into a circle using [[electromagnet]]s. The advantage of circular accelerators over linear accelerators (''linacs'') is that the ring topology allows continuous acceleration, as the particle can transit indefinitely. Another advantage is that a circular accelerator is smaller than a linear accelerator of comparable power (i.e. a linac would have to be extremely long to have the equivalent power of a circular accelerator). |
|||
At lower energies, beams of accelerated nuclei are also used in medicine as [[particle therapy]], for the treatment of cancer. |
|||
DC accelerator types capable of accelerating particles to speeds sufficient to cause nuclear reactions are [[Cockcroft–Walton generator]]s or [[voltage multiplier]]s, which convert AC to high voltage DC, or [[Van de Graaff generator]]s that use static electricity carried by belts. |
|||
=== Radiation sterilization of medical devices === |
|||
[[Electron beam processing]] is commonly used for sterilization. [[Electron beams]] are an on-off technology that provide a much higher dose rate than gamma or X-rays emitted by [[radioisotope]]s like [[cobalt-60]] (<sup>60</sup>Co) or [[caesium-137]] (<sup>137</sup>Cs). Due to the higher dose rate, less exposure time is required and polymer degradation is reduced. Because [[electron]]s carry a charge, electron beams are less penetrating than both gamma and X-rays.<ref>{{cite web|url= https://lss.fnal.gov/archive/2019/conf/fermilab-conf-19-574-di.pdf|title= 2019 Midwest Medical Device Sterilization Workshop: Summary Report |
|||
|date= November 2019|publisher= United States [[United States Department of Energy|Department of Energy]]}}</ref> |
|||
== Electrostatic particle accelerators == |
|||
[[File:Cockcroft–Walton generator.jpg|thumb|150px|A [[Cockcroft–Walton generator]] ([[Philips]], 1937), residing in [[Science Museum (London)]].]] |
|||
{{Main|Electrostatic particle accelerator}} |
|||
[[Image:2mv accelerator-MJC01.jpg|thumb|250px|right|upright=2|A 1960s single stage 2 MeV linear Van de Graaff accelerator, here opened for maintenance]] |
|||
Historically, the first accelerators used simple technology of a single static high voltage to accelerate charged particles. The charged particle was accelerated through an evacuated tube with an electrode at either end, with the static potential across it. Since the particle passed only once through the potential difference, the output energy was limited to the accelerating voltage of the machine. While this method is still extremely popular today, with the electrostatic accelerators greatly out-numbering any other type, they are more suited to lower energy studies owing to the practical voltage limit of about 1 MV for air insulated machines, or 30 MV when the accelerator is operated in a tank of pressurized gas with high [[dielectric strength]], such as [[sulfur hexafluoride]]. In a ''tandem accelerator'' the potential is used twice to accelerate the particles, by reversing the charge of the particles while they are inside the terminal. This is possible with the acceleration of [[atomic nuclei]] by using [[anion]]s (negatively charged [[ion]]s), and then passing the beam through a thin foil to strip electrons off the anions inside the high voltage terminal, converting them to cations (positively charged ions), which are accelerated again as they leave the terminal. |
|||
The two main types of electrostatic accelerator are the [[Cockcroft–Walton accelerator]], which uses a diode-capacitor voltage multiplier to produce high voltage, and the [[Van de Graaff accelerator]], which uses a moving fabric belt to carry charge to the high voltage electrode. Although electrostatic accelerators accelerate particles along a straight line, the term linear accelerator is more often used for accelerators that employ oscillating rather than static electric fields. |
|||
== Electrodynamic (electromagnetic) particle accelerators == |
|||
Due to the high voltage ceiling imposed by electrical discharge, in order to accelerate particles to higher energies, techniques involving dynamic fields rather than static fields are used. Electrodynamic acceleration can arise from either of two mechanisms: non-resonant [[Electromagnetic induction|magnetic induction]], or resonant circuits or [[Microwave cavity|cavities]] excited by oscillating [[radio frequency]] (RF) fields.<ref> |
|||
{{cite book |
|||
|last=Humphries |
|||
|first=Stanley |
|||
|year=1986 |
|||
|url=https://archive.org/details/principlescharge00hump/page/6 |
|||
|title=Principles of Charged Particle Acceleration |
|||
|page=[https://archive.org/details/principlescharge00hump/page/6 6] |
|||
|publisher=[[Wiley-Interscience]] |
|||
|isbn=978-0471878780 |
|||
}}</ref> |
|||
Electrodynamic accelerators can be ''linear'', with particles accelerating in a straight line, or ''circular'', using magnetic fields to bend particles in a roughly circular orbit. |
|||
=== Magnetic induction accelerators === |
|||
Magnetic induction accelerators accelerate particles by induction from an increasing magnetic field, as if the particles were the secondary winding in a transformer. The increasing magnetic field creates a circulating electric field which can be configured to accelerate the particles. Induction accelerators can be either linear or circular. |
|||
==== Linear induction accelerators ==== |
|||
{{Main|Linear induction accelerator}} |
|||
Linear induction accelerators utilize ferrite-loaded, non-resonant induction cavities. Each cavity can be thought of as two large washer-shaped disks connected by an outer cylindrical tube. Between the disks is a ferrite toroid. A voltage pulse applied between the two disks causes an increasing magnetic field which inductively couples power into the charged particle beam.<ref> |
|||
{{cite book |
|||
|last=Humphries |
|||
|first=Stanley |
|||
|year=1986 |
|||
|chapter-url=http://www.fieldp.com/cpa.html |
|||
|chapter=Linear Induction Accelerators |
|||
|title=Principles of Charged Particle Acceleration |
|||
|pages=[https://archive.org/details/principlescharge00hump/page/283 283–325] |
|||
|publisher=[[Wiley-Interscience]] |
|||
|isbn=978-0471878780 |
|||
|url=https://archive.org/details/principlescharge00hump/page/283 |
|||
}}</ref> |
|||
The linear induction accelerator was invented by [[Nicholas Christofilos|Christofilos]] in the 1960s.<ref> |
|||
{{cite book |
|||
|last1=Christofilos |first1=N.C. |
|||
|year=1963 |
|||
|contribution=High-current linear induction accelerator for electrons |
|||
|url=http://inspirehep.net/record/918919/files/HEACC63_II_785-791.pdf |
|||
|title=Proceedings, 4th International Conference on High-Energy Accelerators (HEACC63) |
|||
|pages=1482–1488 |
|||
|display-authors=etal |
|||
}}</ref> Linear induction accelerators are capable of accelerating very high beam currents (>1000 A) in a single short pulse. They have been used to generate X-rays for flash radiography (e.g. [[Dual-Axis Radiographic Hydrodynamic Test Facility|DARHT]] at [[LANL]]), and have been considered as particle injectors for [[magnetic confinement fusion]] and as drivers for [[free electron laser]]s. |
|||
==== Betatrons ==== |
|||
{{Main|Betatron}} |
|||
The [[Betatron]] is a circular magnetic induction accelerator, invented by [[Donald William Kerst|Donald Kerst]] in 1940 for accelerating [[electron]]s. The concept originates ultimately from Norwegian-German scientist [[Rolf Widerøe]].<ref>{{cite book |last=Sørheim |first=Aashild |url=http://link.springer.com/10.1007/978-3-030-26338-6 |title=Obsessed by a Dream: The Physicist Rolf Widerøe – a Giant in the History of Accelerators |date=5 November 2019 |publisher=Springer International Publishing |isbn=978-3-030-26337-9 |series=Springer Biographies |location=Cham |language=en |doi=10.1007/978-3-030-26338-6|s2cid=211929538 }}</ref><ref>Pedro Waloschek (ed.): ''The Infancy of Particle Accelerators: Life and Work of Rolf Wideröe'', Vieweg, 1994</ref> These machines, like synchrotrons, use a donut-shaped ring magnet (see below) with a cyclically increasing B field, but accelerate the particles by induction from the increasing magnetic field, as if they were the secondary winding in a transformer, due to the changing magnetic flux through the orbit.<ref>{{cite book | editor1-last=Chao | editor1-first=A. W. | editor2-last=Mess | editor2-first=K. H. | editor3-last=Tigner | editor3-first=M. |display-editors = 3 | editor4-last=Zimmermann | editor4-first=F. | year=2013 | title=Handbook of Accelerator Physics and Engineering | edition=2nd | publisher=World Scientific | isbn=978-981-4417-17-4 | doi=10.1142/8543 | s2cid=108427390 | url=https://cds.cern.ch/record/384825 }}</ref><ref> |
|||
{{cite book |
|||
|last=Humphries |
|||
|first=Stanley |
|||
|year=1986 |
|||
|chapter-url=http://www.fieldp.com/cpa.html |
|||
|chapter=Betatrons |
|||
|title=Principles of Charged Particle Acceleration |
|||
|page=[https://archive.org/details/principlescharge00hump/page/326 326ff] |
|||
|publisher=[[Wiley-Interscience]] |
|||
|isbn=978-0471878780 |
|||
|url=https://archive.org/details/principlescharge00hump/page/326 |
|||
}}</ref> |
|||
Achieving constant orbital radius while supplying the proper accelerating electric field requires that the magnetic flux linking the orbit be somewhat independent of the magnetic field on the orbit, bending the particles into a constant radius curve. These machines have in practice been limited by the large radiative losses suffered by the electrons moving at nearly the speed of light in a relatively small radius orbit. |
|||
=== Linear accelerators === |
|||
{{Main|Linear particle accelerator}} |
|||
[[File:Desy tesla cavity01.jpg|thumb|Modern [[superconducting radio frequency]], multicell linear accelerator component.]] |
|||
In a [[linear particle accelerator]] (linac), particles are accelerated in a straight line with a target of interest at one end. They are often used to provide an initial low-energy kick to particles before they are injected into circular accelerators. The longest linac in the world is the [[Stanford Linear Accelerator Center|Stanford Linear Accelerator]], SLAC, which is {{convert|3|km|mi|abbr=on}} long. SLAC was originally an [[electron]]–[[positron]] collider but is now a X-ray [[Free-electron laser]]. |
|||
Linear high-energy accelerators use a linear array of plates (or drift tubes) to which an alternating high-energy field is applied. As the particles approach a plate they are accelerated towards it by an opposite polarity charge applied to the plate. As they pass through a hole in the plate, the [[Electrical polarity|polarity]] is switched so that the plate now repels them and they are now accelerated by it towards the next plate. Normally a stream of "bunches" of particles are accelerated, so a carefully controlled AC voltage is applied to each plate to continuously repeat this process for each bunch. |
|||
As the particles approach the speed of light the switching rate of the electric fields becomes so high that they operate at [[radio frequency|radio frequencies]], and so [[microwave cavity|microwave cavities]] are used in higher energy machines instead of simple plates. |
|||
Linear accelerators are also widely used in [[medicine]], for [[radiotherapy]] and [[radiosurgery]]. Medical grade linacs accelerate electrons using a [[klystron]] and a complex bending magnet arrangement which produces a beam of energy {{val|6|–|30|ul=MeV}}. The electrons can be used directly or they can be collided with a target to produce a beam of [[X-rays]]. The reliability, flexibility and accuracy of the radiation beam produced has largely supplanted the older use of [[cobalt-60]] therapy as a treatment tool. |
|||
=== Circular or cyclic RF accelerators <span class="anchor" id="Circular accelerators"></span> === |
|||
<!-- This Anchor tag serves to provide a permanent target for incoming section links. Please do not remove it, nor modify it, except to add another appropriate anchor. If you modify the section title, please anchor the old title. It is always best to anchor an old section header that has been changed so that links to it will not be broken. See [[Template:Anchor]] for details. This text is produced using {{subst:Anchor comment}} --> |
|||
In the circular accelerator, particles move in a circle until they reach enough energy. The particle track is typically bent into a circle using [[electromagnet]]s. The advantage of circular accelerators over linear accelerators (''linacs'') is that the ring topology allows continuous acceleration, as the particle can transit indefinitely. Another advantage is that a circular accelerator is smaller than a linear accelerator of comparable power (i.e. a linac would have to be extremely long to have the equivalent power of a circular accelerator). |
|||
Depending on the energy and the particle being accelerated, circular accelerators suffer a disadvantage in that the particles emit [[synchrotron radiation]]. When any charged particle is accelerated, it emits [[electromagnetic radiation]] and [[secondary emission]]s. As a particle traveling in a circle is always accelerating towards the center of the circle, it continuously radiates towards the tangent of the circle. This radiation is called [[synchrotron light]] and depends highly on the mass of the accelerating particle. For this reason, many high energy electron accelerators are linacs. Certain accelerators ([[synchrotron]]s) are however built specially for producing synchrotron light ([[X-ray]]s). |
Depending on the energy and the particle being accelerated, circular accelerators suffer a disadvantage in that the particles emit [[synchrotron radiation]]. When any charged particle is accelerated, it emits [[electromagnetic radiation]] and [[secondary emission]]s. As a particle traveling in a circle is always accelerating towards the center of the circle, it continuously radiates towards the tangent of the circle. This radiation is called [[synchrotron light]] and depends highly on the mass of the accelerating particle. For this reason, many high energy electron accelerators are linacs. Certain accelerators ([[synchrotron]]s) are however built specially for producing synchrotron light ([[X-ray]]s). |
||
Since the [[special theory of relativity]] requires that matter always travels slower than the speed of light in |
Since the [[special theory of relativity]] requires that matter always travels slower than the speed of light in [[vacuum]], in high-energy accelerators, as the energy increases the particle speed approaches the speed of light as a limit, but never attains it. Therefore, particle physicists do not generally think in terms of speed, but rather in terms of a particle's [[energy]] or [[momentum]], usually measured in [[electron volt]]s (eV). An important principle for circular accelerators, and [[particle beam]]s in general, is that the [[curvature]] of the particle trajectory is proportional to the particle charge and to the magnetic field, but inversely proportional to the (typically [[special relativity|relativistic]]) [[momentum]]. |
||
An important principle for circular accelerators, and [[particle beam]]s in general, is that the [[curvature]] of the particle trajectory is proportional to the particle charge and to the magnetic field, but inversely proportional to the (typically [[special relativity|relativistic]]) [[momentum]]. |
|||
===Cyclotrons=== |
==== Cyclotrons ==== |
||
[[File:Berkeley 60-inch cyclotron.jpg|thumb|Lawrence's 60 inch cyclotron, with magnet poles 60 inches (5 feet, 1.5 meters) in diameter, at the [[University of California]] [[Lawrence Radiation Laboratory]], Berkeley, in August, 1939, the most powerful accelerator in the world at the time. [[Glenn T. Seaborg]] and [[Edwin McMillan]] ''(right)'' used it to discover [[plutonium]], [[neptunium]] and many other transuranic elements and isotopes, for which they received the 1951 [[Nobel Prize]] in chemistry.]] |
|||
The earliest circular accelerators were [[cyclotron]]s, invented in 1929 by [[Ernest O. Lawrence]] at the [[University of California, Berkeley]]. Cyclotrons have a single pair of hollow 'D'-shaped plates to accelerate the particles and a single large [[dipole magnet]] to bend their path into a circular orbit. |
|||
{{Main|Cyclotron}} |
|||
It is a characteristic property of charged particles in a uniform and constant magnetic field B that they orbit with a constant period, at a frequency called the [[cyclotron frequency]], so long as their speed is small compared to the speed of light ''c''. |
|||
The earliest operational circular accelerators were [[cyclotron]]s, invented in 1929 by [[Ernest Lawrence]] at the [[University of California, Berkeley]]. Cyclotrons have a single pair of hollow D-shaped plates to accelerate the particles and a single large [[dipole magnet]] to bend their path into a circular orbit. It is a characteristic property of charged particles in a uniform and constant magnetic field B that they orbit with a constant period, at a frequency called the [[cyclotron frequency]], so long as their speed is small compared to the speed of light ''c''. This means that the accelerating D's of a cyclotron can be driven at a constant frequency by a RF accelerating power source, as the beam spirals outwards continuously. The particles are injected in the center of the magnet and are extracted at the outer edge at their maximum energy. |
|||
This means that the accelerating D's of a cyclotron can be driven at a constant frequency by a radio frequency (RF) accelerating power source, as the beam spirals outwards continuously. |
|||
The particles are injected in the centre of the magnet and are extracted at the outer edge at their maximum energy. |
|||
Cyclotrons reach an energy limit because of [[relativistic mass|relativistic effects]] whereby the particles effectively become more massive, so that their cyclotron frequency drops out of sync with the accelerating RF. Therefore, simple cyclotrons can accelerate protons only to an energy of around 15 million electron volts (15 MeV, corresponding to a speed of roughly 10% of ''c''), because the protons get out of phase with the driving electric field. If accelerated further, the beam would continue to spiral outward to a larger radius but the particles would no longer gain enough speed to complete the larger circle in step with the accelerating RF. To accommodate relativistic effects the magnetic field needs to be increased to higher radii as is done in [[isochronous cyclotron]]s. An example of an isochronous cyclotron is the [[Paul Scherrer Institute|PSI Ring cyclotron]] in Switzerland, which provides protons at the energy of 590 MeV which corresponds to roughly 80% of the speed of light. The advantage of such a cyclotron is the maximum achievable extracted proton current which is currently 2.2 mA. The energy and current correspond to 1.3 MW beam power which is the highest of any accelerator currently existing. |
|||
Cyclotrons reach an energy limit because of [[relativistic mass|relativistic effects]] whereby the particles effectively become more massive, so that their cyclotron frequency drops out of synch with the accelerating RF. |
|||
Therefore simple cyclotrons can accelerate protons only to an energy of around 15 million electron volts (15 MeV, corresponding to a speed of roughly 10% of ''c''), because the protons get out of phase with the driving electric field. |
|||
If accelerated further, the beam would continue to spiral outward to a larger radius but the particles would no longer gain enough speed to complete the larger circle in step with the accelerating RF. |
|||
Cyclotrons are nevertheless still useful for lower energy applications. |
|||
===Synchrocyclotrons and isochronous cyclotrons=== |
==== Synchrocyclotrons and isochronous cyclotrons ==== |
||
{{ |
{{Main|Synchrocyclotron|Isochronous cyclotron}} |
||
[[ |
[[File:Orsay proton therapy dsc04444.jpg|thumb|A magnet in the synchrocyclotron at the [[Orsay]] [[proton therapy]] center]] |
||
A classic cyclotron can be modified to increase its energy limit. The historically first approach was the [[synchrocyclotron]], which accelerates the particles in bunches. It uses a constant [[magnetic field]] <math>B</math>, but reduces the accelerating field's frequency so as to keep the particles in step as they spiral outward, matching their mass-dependent [[cyclotron resonance]] frequency. This approach suffers from low average beam intensity due to the bunching, and again from the need for a huge magnet of large radius and constant field over the larger orbit demanded by high energy. |
|||
There are ways of modifying the classic cyclotron to increase the energy limit. |
|||
This may be done in a continuous beam, constant frequency, machine by shaping the magnet poles so to increase magnetic field with radius. |
|||
Then higher energy particles travel a shorter distance in each orbit than they otherwise would, and can remain in phase with the accelerating field. |
|||
Such machines are called isochronous cyclotrons. Their advantage is that they can deliver continuous beams of higher average intensity, which is useful for some applications. |
|||
The main disadvantages are the size and cost of the large magnet needed, and the difficulty in achieving the higher field required at the outer edge. |
|||
The second approach to the problem of accelerating relativistic particles is the [[isochronous cyclotron]]. In such a structure, the accelerating field's frequency (and the cyclotron resonance frequency) is kept constant for all energies by shaping the magnet poles so to increase magnetic field with radius. Thus, all particles get accelerated in [[isochronous]] time intervals. Higher energy particles travel a shorter distance in each orbit than they would in a classical cyclotron, thus remaining in phase with the accelerating field. The advantage of the isochronous cyclotron is that it can deliver continuous beams of higher average intensity, which is useful for some applications. The main disadvantages are the size and cost of the large magnet needed, and the difficulty in achieving the high magnetic field values required at the outer edge of the structure. |
|||
Another possibility, the synchrocyclotron, accelerates the particles in bunches, in a constant B field, but reduces the RF accelerating field's frequency so as to keep the particles in step as they spiral outward. This approach suffers from low average beam intensity due to the bunching, and again from the need for a huge magnet of large radius and constant field over the larger orbit demanded by high energy. |
|||
Synchrocyclotrons have not been built since the isochronous cyclotron was developed. |
|||
===Betatrons=== |
|||
{{main|Betatron}} |
|||
Another type of circular accelerator, invented in 1940 for accelerating [[electron]]s, is the [[Betatron]]. |
|||
These machines, like synchrotrons, use a donut-shaped ring magnet (see below) with a cyclically increasing B field, but accelerate the particles by induction from the increasing magnetic field, as if they were the secondary winding in a transformer, |
|||
due to the changing magnetic flux through the orbit. |
|||
Achieving constant orbital radius while supplying the proper accelerating electric field requires that the magnetic flux linking the orbit be somewhat independent of the magnetic field on the orbit, bending the particles into a constant radius curve. |
|||
These machines have in practice been limited by the large radiative losses suffered by the electrons moving at nearly the speed of light in a relatively small radius orbit. |
|||
===Synchrotrons=== |
==== Synchrotrons ==== |
||
{{ |
{{Main|Synchrotron}} |
||
[[ |
[[File:Fermilab.jpg|thumb|Aerial photo of the [[Tevatron]] (background ring) and Main Injector (foreground ring which is not actually circular) rings at [[Fermilab]]. The Tevatron ring also contains Main Ring and a section of it is still used for downstream experiments. The Main Injector below (about half the diameter of the Tevatron) is for preliminary acceleration, beam cooling and storage, etc.]] |
||
To reach still higher energies, with relativistic mass approaching or exceeding the rest mass of the particles (for protons, billions of electron volts or [[GeV]]), it is necessary to use a [[synchrotron]]. This is an accelerator in which the particles are accelerated in a ring of constant radius. An immediate advantage over cyclotrons is that the magnetic field need only be present over the actual region of the particle orbits, which is much narrower than that of the ring. (The largest cyclotron built in the US had a {{convert|184|in|m|adj=mid|-diameter}} magnet pole, whereas the diameter of synchrotrons such as the [[LEP]] and [[Large Hadron Collider|LHC]] is nearly 10 km. The aperture of the two beams of the LHC is of the order of a centimeter.) The LHC contains 16 RF cavities, 1232 superconducting dipole magnets for beam steering, and 24 quadrupoles for beam focusing.<ref name=CERN-MAG>["Pulling together: Superconducting electromagnets" CERN; https://home.cern/science/engineering/pulling-together-superconducting-electromagnets {{Webarchive|url=https://web.archive.org/web/20200423135126/https://home.cern/science/engineering/pulling-together-superconducting-electromagnets |date=2020-04-23 }}]</ref> Even at this size, the LHC is limited by its ability to steer the particles without them going adrift. This limit is theorized to occur at 14 TeV.<ref name=CERN-13>["Restarting the LHC: Why 13 TeV?" CERN; https://home.cern/science/engineering/restarting-lhc-why-13-tev {{Webarchive|url=https://web.archive.org/web/20181007130734/https://home.cern/about/engineering/restarting-lhc-why-13-tev |date=2018-10-07 }}]</ref> |
|||
To reach still higher energies, with relativistic mass approaching or exceeding the rest mass of the particles (for protons, billions of electron volts [[GeV]]), it is necessary to use a [[synchrotron]]. This is an accelerator in which the particles are accelerated in a ring of constant radius. |
|||
An immediate advantage over cyclotrons is that the magnetic field need only be present over the actual region of the particle orbits, which is very much narrower than the diameter of the ring. |
|||
(The largest cyclotron built in the US had a 184 inch diameter magnet pole, whereas the diameter of the [[LEP]] and [[Large Hadron Collider|LHC]] is nearly 10 km. The aperture of the beam of the latter is of the order of centimeters.) |
|||
However, since the particle momentum increases during acceleration, it is necessary to turn up the magnetic field B in proportion to maintain constant curvature of the orbit. |
However, since the particle momentum increases during acceleration, it is necessary to turn up the magnetic field B in proportion to maintain constant curvature of the orbit. In consequence, synchrotrons cannot accelerate particles continuously, as cyclotrons can, but must operate cyclically, supplying particles in bunches, which are delivered to a target or an external beam in beam "spills" typically every few seconds. |
||
In consequence synchrotrons cannot accelerate particles continuously, as cyclotrons can, but must operate cyclically, supplying particles in bunches, which are delivered to a target or an external beam in beam "spills" typically every few seconds. |
|||
Since high energy synchrotrons do most of their work on particles that are already traveling at nearly the speed of light ''c'', the time to complete one orbit of the ring is nearly constant, as is the frequency of the [[Cavity resonator|RF cavity resonators]] used to drive the acceleration. |
Since high energy synchrotrons do most of their work on particles that are already traveling at nearly the speed of light ''c'', the time to complete one orbit of the ring is nearly constant, as is the frequency of the [[Cavity resonator|RF cavity resonators]] used to drive the acceleration. |
||
In modern synchrotrons, the beam aperture is small and the magnetic field does not cover the entire area of the particle orbit as it does for a cyclotron, so several necessary functions can be separated. Instead of one huge magnet, one has a line of hundreds of bending magnets, enclosing (or enclosed by) vacuum connecting pipes. The design of synchrotrons was revolutionized in the early 1950s with the discovery of the [[strong focusing]] concept.<ref>{{Cite journal | last1 = Courant | first1 = E. D. | author-link1 = Ernest Courant| last2 = Livingston | first2 = M. S. | author-link2 = Milton Stanley Livingston| last3 = Snyder | first3 = H. S. | author-link3 = Hartland Sweet Snyder| doi = 10.1103/PhysRev.88.1190 | bibcode = 1952PhRv...88.1190C| title = The Strong-Focusing Synchrotron—A New High Energy Accelerator | journal = [[Physical Review]] | volume = 88 | issue = 5 | pages = 1190–1196| year = 1952 | hdl = 2027/mdp.39015086454124 | hdl-access = free }}</ref><ref>{{Cite journal | last1 = Blewett | first1 = J. P.| title = Radial Focusing in the Linear Accelerator | doi = 10.1103/PhysRev.88.1197 | bibcode = 1952PhRv...88.1197B| journal = [[Physical Review]] | volume = 88 | issue = 5 | pages = 1197–1199| year = 1952 }}</ref><ref>{{Cite web|title=The Alternating Gradient Concept|url=http://www.bnl.gov/bnlweb/history/focusing.asp|publisher=[[Brookhaven National Laboratory]]|access-date=2009-04-29|archive-date=2013-04-02|archive-url=https://web.archive.org/web/20130402193938/http://www.bnl.gov/bnlweb/history/focusing.asp|url-status=dead}}</ref> The focusing of the beam is handled independently by specialized [[quadrupole magnets]], while the acceleration itself is accomplished in separate RF sections, rather similar to short linear accelerators.<ref>{{cite journal |last1=Efimov |first1=S.P. |last2=Korenev |first2=I.L. |last3=Yudin |first3=L.A. |title=Resonances of electron beam focused by a helical quadrupole magnetic field |journal=Radiophysics and Quantum Electronics |date=1990 |volume=33 |issue=1 |pages=88–95 |doi=10.1007/BF01037825 |s2cid=123706289 }}</ref> Also, there is no necessity that cyclic machines be circular, but rather the beam pipe may have straight sections between magnets where beams may collide, be cooled, etc. This has developed into an entire separate subject, called "beam physics" or "beam optics".<ref>{{Cite web|title=World of Beams Homepage|url=http://bc1.lbl.gov/CBP_pages/educational/WoB/home.htm|publisher=[[Lawrence Berkeley National Laboratory]]|access-date=2009-04-29|archive-url=https://web.archive.org/web/20050302091712/http://bc1.lbl.gov/CBP_pages/educational/WoB/home.htm|archive-date=2005-03-02|url-status=dead}}</ref> |
|||
Note also a further point about modern synchrotrons: because the beam aperture is small and the magnetic field does not cover the entire area of the particle orbit as it does for a cyclotron, several necessary functions can be separated. |
|||
Instead of one huge magnet, one has a line of hundreds of bending magnets, enclosing (or enclosed by) vacuum connecting pipes. |
|||
The design of synchrotrons was revolutionized in the early 1950s with the discovery of the [[strong focusing]] concept.<ref>http://prola.aps.org/abstract/PR/v88/i5/p1190_1 Courant, Livingston and Snyder, The Strong-Focusing Synchroton—A New High Energy Accelerator</ref><ref>http://prola.aps.org/abstract/PR/v88/i5/p1197_1 Blewett, Radial Focusing in the Linear Accelerator</ref><ref>{{cite web|url=http://www.bnl.gov/bnlweb/history/focusing.asp|title=BNL page on strong focusing.}}</ref> |
|||
The focusing of the beam is handled independently by specialized [[quadrupole magnets]], while the acceleration itself is accomplished in separate RF sections, rather similar to short linear accelerators. |
|||
Also, there is no necessity that cyclic machines be circular, but rather the beam pipe may have straight sections between magnets where beams may collide. be cooled, etc. |
|||
This has developed into an entire separate subject, called "beam physics" or "beam optics".<ref>{{cite web|url=http://bc1.lbl.gov/CBP_pages/educational/WoB/home.htm|title=Lawrence Berkeley National Laboratory: World of Beams}}</ref> |
|||
More complex modern synchrotrons such as the |
More complex modern synchrotrons such as the Tevatron, [[LEP]], and LHC may deliver the particle bunches into [[storage ring]]s of magnets with a constant magnetic field, where they can continue to orbit for long periods for experimentation or further acceleration. The highest-energy machines such as the Tevatron and LHC are actually accelerator complexes, with a cascade of specialized elements in series, including linear accelerators for initial beam creation, one or more low energy synchrotrons to reach intermediate energy, storage rings where beams can be accumulated or "cooled" (reducing the magnet aperture required and permitting tighter focusing; see [[Particle beam cooling|beam cooling]]), and a last large ring for final acceleration and experimentation. |
||
The highest-energy machines such as the Tevatron and LHC are actually accelerator complexes, with a cascade of specialized elements in series, including linear accelerators for initial beam creation, one or more low energy synchrotrons to reach intermediate energy, storage rings where beams can be accumulated or "cooled" (reducing the magnet aperture required and permitting tighter focusing; see [[stochastic cooling|beam cooling]]), and a last large ring for final acceleration and experimentation. |
|||
[[File:DESY1.jpg|thumb|Segment of an electron synchrotron at [[DESY]]]] |
|||
====Electron synchrotrons==== |
|||
[[Image:DESY1.jpg|thumb|Segment of an electron synchrotron at [[DESY]]]] |
|||
Circular electron accelerators fell somewhat out of favor for particle physics around the time that [[SLAC]] was constructed, because their synchrotron losses were considered economically prohibitive and because their beam intensity was lower than for the unpulsed linear machines. |
|||
The Cornell Electron Synchrotron, built at low cost in the late 1960s, was the first in a series of high-energy circular electron accelerators built for fundamental particle physics, culminating in the [[LEP]] at CERN. |
|||
===== Electron synchrotrons ===== |
|||
A large number of electron synchrotrons have been built in the past two decades, specialized to be synchrotron light sources, of ultraviolet light and X rays; see below. |
|||
{{See also|Synchrotron light source}} |
|||
Circular electron accelerators fell somewhat out of favor for particle physics around the time that [[SLAC]]'s linear particle accelerator was constructed, because their synchrotron losses were considered economically prohibitive and because their beam intensity was lower than for the unpulsed linear machines. The [[Cornell Laboratory for Accelerator-based Sciences and Education|Cornell Electron Synchrotron]], built at low cost in the late 1970s, was the first in a series of high-energy circular electron accelerators built for fundamental particle physics, the last being [[LEP]], built at CERN, which was used from 1989 until 2000. |
|||
===Storage rings=== |
|||
{{main|Storage ring}} |
|||
For some applications, it is useful to store beams of high energy particles for some time (with modern high [[vacuum]] technology, up to many hours) without further acceleration. |
|||
This is especially true for [[collider|colliding beam accelerators]], in which two beams moving in opposite directions are made to collide with each other, with a large gain in effective collision energy. |
|||
Because relatively few collisions occur at each pass through the intersection point of the two beams, it is customary to first accelerate the beams to the desired energy, and then store them in storage rings, which are essentially synchrotron rings of magnets, with no significant RF power for acceleration. |
|||
A large number of electron synchrotrons have been built in the past two decades, as part of [[synchrotron light source]]s that emit ultraviolet light and X rays; see below. |
|||
===Synchrotron radiation sources=== |
|||
{{main|Synchrotron light sources}} |
|||
==== Synchrotron radiation sources ==== |
|||
Some circular accelerators have been built to deliberately generate radiation (called [[synchrotron light]]) as [[X-rays]] also called synchrotron radiation, for example the [[Diamond Light Source]] being built at the [[Rutherford Appleton Laboratory]] in [[England]] or the [[Advanced Photon Source]] at [[Argonne National Laboratory]] in [[Illinois]], USA. High-energy X-rays are useful for [[X-ray spectroscopy]] of [[protein]]s or [[X-ray absorption fine structure]] (XAFS) for example. |
|||
{{Main|Synchrotron light sources}} |
|||
Some circular accelerators have been built to deliberately generate radiation (called [[synchrotron light]]) as [[X-rays]] also called synchrotron radiation, for example the [[Diamond Light Source]] which has been built at the [[Rutherford Appleton Laboratory]] in England or the [[Advanced Photon Source]] at [[Argonne National Laboratory]] in [[Illinois]], USA. High-energy X-rays are useful for [[X-ray spectroscopy]] of [[protein]]s or [[X-ray absorption fine structure]] (XAFS), for example. |
|||
Synchrotron radiation is more powerfully emitted by lighter particles, so these accelerators are invariably [[electron]] accelerators. Synchrotron radiation allows for better imaging as researched and developed at [[Stanford Linear Accelerator Center|SLAC's SPEAR]]. |
Synchrotron radiation is more powerfully emitted by lighter particles, so these accelerators are invariably [[electron]] accelerators. Synchrotron radiation allows for better imaging as researched and developed at [[Stanford Linear Accelerator Center|SLAC's SPEAR]]. |
||
==== Fixed-field alternating gradient accelerators ==== |
|||
===History=== |
|||
{{Main|Fixed-Field alternating gradient Accelerator}} |
|||
{{main|List of accelerators in particle physics}} |
|||
[[Fixed-Field alternating gradient Accelerator|Fixed-Field Alternating Gradient accelerators (FFA)]]s, in which a magnetic field which is fixed in time, but with a radial variation to achieve [[strong focusing]], allows the beam to be accelerated with a high repetition rate but in a much smaller radial spread than in the cyclotron case. Isochronous FFAs, like isochronous cyclotrons, achieve continuous beam operation, but without the need for a huge dipole bending magnet covering the entire radius of the orbits. Some new developments in FFAs are covered in.<ref> |
|||
Lawrence's first cyclotron was a mere 4 inches (100 mm) in diameter. |
|||
{{Cite journal|title=The Next Big Beam?|last=Clery|first=D.|year=2010|journal=[[Science (journal)|Science]]|volume=327 |issue=5962|pages=142–144|doi=10.1126/science.327.5962.142|pmid=20056871|bibcode = 2010Sci...327..142C}}</ref> |
|||
Later he built a machine with a 60 in [[dipole]] face, and planned one with a |
|||
[[List of accelerators in particle physics|184-inch]] dia, which was, however, taken over for [[World War II]]-related work connected with uranium [[isotope separation]]; after the war it continued in service for research and medicine over many years. |
|||
==== Rhodotron ==== |
|||
The first large proton [[synchrotron]] was the [[Cosmotron]] at [[Brookhaven National Laboratory]], which accelerated [[protons]] to about 3 [[GeV]]. |
|||
[[File:Rhodotron.svg|thumb|A diagram of a Rhodotron. The electron beam is in red. E is the electron gun, L is an electron lens, C is the radiofrequency cavity, and M is a bending magnet.]] |
|||
The [[Bevatron]] at Berkeley, completed in 1954, was specifically designed to accelerate protons to sufficient energy to create [[antiprotons]], and verify the [[antimatter|particle-antiparticle symmetry]] of nature, then only strongly suspected. |
|||
A Rhodotron is an industrial electron accelerator first proposed in 1987 by J. Pottier of the [[French Alternative Energies and Atomic Energy Commission|French Atomic |
|||
The [[Alternating Gradient Synchrotron]] (AGS) at Brookhaven was the first large synchrotron with alternating gradient, "[[strong focusing]]" magnets, which greatly reduced the required aperture of the beam, and correspondingly the size and cost of the bending magnets. |
|||
Energy Agency (CEA)]],<ref>{{Cite book|url=https://books.google.com/books?id=W4C6CgAAQBAJ&dq=rhodotron+pottier&pg=PA55|title=Handbook of Accelerator Physics and Engineering|first1=Alexander Wu|last1=Chao|first2=Karl Hubert|last2=Mess|date=December 31, 2013|publisher=World Scientific|isbn=978-981-4415-85-9 |via=Google Books}}</ref> manufactured by Belgian company [[Ion Beam Applications]]. It accelerates electrons by recirculating them across the diameter of a cylinder-shaped radiofrequency cavity. A Rhodotron has an electron gun, which emits an electron beam that is attracted to a pillar in the center of the cavity. The pillar has holes the electrons can pass through. The electron beam passes through the pillar via one of these holes and then travels through a hole in the wall of the cavity, and meets a bending magnet, the beam is then bent and sent back into the cavity, to another hole in the pillar, the electrons then again go across the pillar and pass though another part of the wall of the cavity and into another bending magnet, and so on, gradually increasing the energy of the beam until it is allowed to exit the cavity for use. The cylinder and pillar may be lined with copper on the inside.<ref>{{cite book | url=https://books.google.com/books?id=-GO6dGnLbywC&dq=rhodotron&pg=PA216 | isbn=978-981-4383-98-1 | title=Reviews of Accelerator Science and Technology: Accelerator Applications in Industry and the Environment | date=20 February 2012 | publisher=World Scientific }}</ref><ref>{{cite book | url=https://books.google.com/books?id=Kp3FCgAAQBAJ&dq=rhodotron&pg=PA114 | isbn=978-981-4434-61-4 | title=Industrial Accelerators and Their Applications | date=27 June 2012 | publisher=World Scientific }}</ref><ref>{{cite journal | doi=10.1016/0168-583X(94)95146-2 | title=The Rhodotron, a new high-energy, high-power, CW electron accelerator | year=1994 | last1=Jongen | first1=Y. | last2=Abs | first2=M. | last3=Capdevila | first3=J.M. | last4=Defrise | first4=D. | last5=Genin | first5=F. | last6=Nguyen | first6=A. | journal=Nuclear Instruments and Methods in Physics Research Section B | volume=89 | issue=1–4 | pages=60–64 | bibcode=1994NIMPB..89...60J }}</ref> |
|||
The [[Proton Synchrotron]], built at [[CERN]], was the first major European particle accelerator and generally similar to the AGS. |
|||
==== History ==== |
|||
The [[Fermi National Accelerator Laboratory|Fermilab]] [[Tevatron]] has a ring with a beam path of 4 miles (6 km). |
|||
{{Main|List of accelerators in particle physics}} |
|||
The largest circular accelerator ever built was the [[Large Electron Positron|LEP]] [[synchrotron]] at CERN with a circumference 26.6 kilometers, which was an electron/[[positron]] collider. It has been dismantled and the underground tunnel is being reused to implement the [[Large Hadron Collider|LHC]], which is a proton collider. The LHC suffered some delays in its startup in 2008.<ref>{{cite web |
|||
Ernest Lawrence's first cyclotron was a mere 4 inches (100 mm) in diameter. Later, in 1939, he built a machine with a 60-inch diameter pole face, and planned one with a [[List of accelerators in particle physics|184-inch]] diameter in 1942, which was, however, taken over for [[World War II]]-related work connected with uranium [[isotope separation]]; after the war it continued in service for research and medicine over many years. |
|||
| url = http://en.wikinews.org/wiki/Large_Hadron_Collider_damaged,_to_be_shut_down_for_repairs |
|||
| title = Large Hadron Collider damaged, to be shut down for repairs |
|||
The first large proton [[synchrotron]] was the [[Cosmotron]] at [[Brookhaven National Laboratory]], which accelerated [[protons]] to about 3 [[GeV]] (1953–1968). The [[Bevatron]] at Berkeley, completed in 1954, was specifically designed to accelerate protons to enough energy to create [[antiprotons]], and verify the [[antimatter|particle–antiparticle symmetry]] of nature, then only theorized. The [[Alternating Gradient Synchrotron]] (AGS) at Brookhaven (1960–) was the first large synchrotron with alternating gradient, "[[strong focusing]]" magnets, which greatly reduced the required aperture of the beam, and correspondingly the size and cost of the bending magnets. The [[Proton Synchrotron]], built at [[CERN]] (1959–), was the first major European particle accelerator and generally similar to the AGS. |
|||
}}</ref><ref>{{cite web |
|||
| url = http://www.scientificamerican.com/blog/60-second-science/post.cfm?id=lhc-helium-leak-will-shut-collider-2008-09-20 |
|||
| title = LCH helium leak will shut collider | date = 2008-09-20 |
|||
}}</ref> |
|||
The [[Stanford Linear Accelerator]], SLAC, became operational in 1966, accelerating electrons to 30 GeV in a 3 km long waveguide, buried in a tunnel and powered by hundreds of large [[klystron]]s. It is still the largest linear accelerator in existence, and has been upgraded with the addition of storage rings and an electron-positron collider facility. It is also an X-ray and UV synchrotron photon source. |
|||
An apparent later report by the [[BBC]] said "On Friday, a failure, known as a [[quench]], caused around 100 of the LHC's [[Supercooling |super-cooled magnets]] to heat up by as much as 100 degrees." This was later described as a "massive magnet quench".<ref>{{cite web|url=http://news.bbc.co.uk/2/hi/science/nature/7626944.stm|title=news.bbc.co.uk/2/hi/science/nature/7626944.stm<!--INSERT TITLE-->}}</ref><ref>{{cite web|url=http://news.bbc.co.uk/2/hi/science/nature/7627631.stm|title=news.bbc.co.uk/2/hi/science/nature/7627631.stm<!--INSERT TITLE-->}}</ref> |
|||
The [[Fermi National Accelerator Laboratory|Fermilab]] [[Tevatron]] has a ring with a beam path of {{Convert|4|mi|km}}. It has received several upgrades, and has functioned as a proton-antiproton collider until it was shut down due to budget cuts on September 30, 2011. The largest circular accelerator ever built was the [[Large Electron–Positron Collider|LEP]] [[synchrotron]] at CERN with a circumference 26.6 kilometers, which was an electron/[[positron]] collider. It achieved an energy of 209 GeV before it was dismantled in 2000 so that the tunnel could be used for the [[Large Hadron Collider]] (LHC). The LHC is a proton collider, and currently the world's largest and highest-energy accelerator, achieving 6.5 TeV energy per beam (13 TeV in total). |
|||
This caused a rupture of a high magnitude{{ndash}} leaking one ton of liquid helium into the LHC tunnels.<ref>{{cite web|url=http://news.bbc.co.uk/2/hi/science/nature/7627631.stm|title=news.bbc.co.uk/2/hi/science/nature/7627631.stm<!--INSERT TITLE-->}}</ref> The liquid helium is used for more efficient use of power and the super cooling of the [[liquid helium]] allows the [[electrical resistance]] of the [[superconducting magnets]] to be nonexistent{{ndash}} zero [[ohms]]. According to the BBC, before the accident its operating temperature was 1.9 [[kelvins]] (−271 °C; −456 °F)—which is colder than deep space. |
|||
The aborted [[Superconducting Super Collider]] (SSC) in [[Texas]] would have had a circumference of 87 km. |
The aborted [[Superconducting Super Collider]] (SSC) in [[Texas]] would have had a circumference of 87 km. Construction was started in 1991, but abandoned in 1993. Very large circular accelerators are invariably built in tunnels a few metres wide to minimize the disruption and cost of building such a structure on the surface, and to provide shielding against intense secondary radiations that occur, which are extremely penetrating at high energies. |
||
Construction was started in 1991, but abandoned in 1993. |
|||
Very large circular accelerators are invariably built in underground tunnels a few metres wide to minimize the disruption and cost of building such a structure on the surface, and to provide shielding against intense secondary radiations that may occur. |
|||
These are extremely penetrating at high energies. |
|||
Current accelerators such as the [[Spallation Neutron Source]], incorporate superconducting [[cryomodule]]s. The [[Relativistic Heavy Ion Collider]], and [[Large Hadron Collider]] also make use of [[superconductivity|superconducting]] magnets and [[Cavity resonator|RF cavity resonators]] to accelerate particles. |
Current accelerators such as the [[Spallation Neutron Source]], incorporate superconducting [[cryomodule]]s. The [[Relativistic Heavy Ion Collider]], and [[Large Hadron Collider]] also make use of [[superconductivity|superconducting]] magnets and [[Cavity resonator|RF cavity resonators]] to accelerate particles. |
||
== |
== Targets == |
||
The output of a particle accelerator can generally be directed towards multiple lines of experiments, one at a given time, by means of a deviating [[electromagnet]]. This makes it possible to operate multiple experiments without needing to move things around or shutting down the entire accelerator beam. Except for synchrotron radiation sources, the purpose of an accelerator is to generate high-energy particles for interaction with matter. |
The output of a particle accelerator can generally be directed towards multiple lines of experiments, one at a given time, by means of a deviating [[electromagnet]]. This makes it possible to operate multiple experiments without needing to move things around or shutting down the entire accelerator beam. Except for synchrotron radiation sources, the purpose of an accelerator is to generate high-energy particles for interaction with matter. |
||
Line 194: | Line 301: | ||
For synchrotrons, the situation is more complex. Particles are accelerated to the desired energy. Then, a fast acting dipole magnet is used to switch the particles out of the circular synchrotron tube and towards the target. |
For synchrotrons, the situation is more complex. Particles are accelerated to the desired energy. Then, a fast acting dipole magnet is used to switch the particles out of the circular synchrotron tube and towards the target. |
||
A variation commonly used for [[particle physics]] research is a [[collider]], also called a ''storage ring collider''. Two circular synchrotrons are built in close proximity{{ndash}} |
A variation commonly used for [[particle physics]] research is a [[collider]], also called a ''storage ring collider''. Two circular synchrotrons are built in close proximity{{spaced ndash}}usually on top of each other and using the same magnets (which are then of more complicated design to accommodate both beam tubes). Bunches of particles travel in opposite directions around the two accelerators and collide at intersections between them. This can increase the energy enormously; whereas in a fixed-target experiment the energy available to produce new particles is proportional to the square root of the beam energy, in a collider the available energy is linear. |
||
== Detectors NA == |
|||
The detectors gather clues about the particles including their speed and charge. Using these, the scientists can actually work on the particle. The process of detection is very complex it requires strong [[electromagnet]]s and accelerators to generate enough usable information. {{empty section|date=September 2024}} |
|||
== Higher energies == |
|||
<!-- Deleted image removed: [[File:Particle Accelerator Livingston Chart 2010.png|thumb|400px|right|A [[Milton S. Livingston|Livingston]] chart depicting progress in collision energy through 2010. The LHC is the largest collision energy to date, but also represents the first break in the [[log-linear]] trend. {{FFDC|Particle Accelerator Livingston Chart 2010.png|log=2022 June 4}}]] --> |
|||
At present the highest energy accelerators are all circular colliders, but both hadron accelerators and electron accelerators are running into limits. Higher energy hadron and ion cyclic accelerators will require accelerator tunnels of larger physical size due to the increased [[Rigidity (electromagnetism)|beam rigidity]]. |
|||
For cyclic electron accelerators, a limit on practical bend radius is placed by synchrotron radiation losses and the next generation will probably be linear accelerators 10 times the current length. An example of such a next generation electron accelerator is the proposed 40 km long [[International Linear Collider]]. |
|||
It is believed that [[plasma acceleration|plasma wakefield acceleration]] in the form of electron-beam "afterburners" and standalone laser pulsers might be able to provide dramatic increases in efficiency over RF accelerators within two to three decades. In plasma wakefield accelerators, the beam cavity is filled with a plasma (rather than vacuum). A short pulse of electrons or laser light either constitutes or immediately precedes the particles that are being accelerated. The pulse disrupts the plasma, causing the charged particles in the plasma to integrate into and move toward the rear of the bunch of particles that are being accelerated. This process transfers energy to the particle bunch, accelerating it further, and continues as long as the pulse is coherent.<ref>{{cite journal |last=Wright |first=M. E. |date=April 2005 |url=http://www.symmetrymag.org/cms/?pid=1000091 |title=Riding the Plasma Wave of the Future |journal=[[Symmetry Magazine]] |volume=2 |issue=3 |page=12 |access-date=2005-11-10 |archive-url=https://web.archive.org/web/20061002184158/http://www.symmetrymag.org/cms/?pid=1000091 |archive-date=2006-10-02 |url-status=dead }}</ref> |
|||
Energy gradients as steep as 200 GeV/m have been achieved over millimeter-scale distances using laser pulsers<ref>{{cite journal |last=Briezman |first=B. N. |title=Self-Focused Particle Beam Drivers for Plasma Wakefield Accelerators |journal=AIP Conference Proceedings |volume=396 |pages=75–88 |url=http://peaches.ph.utexas.edu/ifs/ifsreports/Self-focused762.pdf |access-date=2005-05-13 |display-authors=etal |bibcode=1997AIPC..396...75B |year=1997 |doi=10.1063/1.52975 |archive-url=https://web.archive.org/web/20050523143908/http://peaches.ph.utexas.edu/ifs/ifsreports/Self-focused762.pdf |archive-date=2005-05-23 |url-status=dead }}</ref> and gradients approaching 1 GeV/m are being produced on the multi-centimeter-scale with electron-beam systems, in contrast to a limit of about 0.1 GeV/m for radio-frequency acceleration alone. Existing electron accelerators such as [[SLAC]] could use electron-beam afterburners to greatly increase the energy of their particle beams, at the cost of beam intensity. Electron systems in general can provide tightly collimated, reliable beams; laser systems may offer more power and compactness. Thus, plasma wakefield accelerators could be used – if technical issues can be resolved – to both increase the maximum energy of the largest accelerators and to bring high energies into university laboratories and medical centres. |
|||
Higher than 0.25 GeV/m gradients have been achieved by a dielectric laser accelerator,<ref>{{cite journal|title=Demonstration of electron acceleration in a laser-driven dielectric microstructure|journal=Nature|volume=503|issue=7474|pages=91–94|last=Peralta|first=E. A.|display-authors=etal|doi=10.1038/nature12664|pmid = 24077116|year=2013|bibcode=2013Natur.503...91P|s2cid=4467824}}</ref> which may present another viable approach to building compact high-energy accelerators.<ref>{{Cite journal |title= Conceptual layout for a wafer-scale dielectric laser accelerator |volume= 1777 |pages= 060002 |doi=10.1063/1.4965631|journal = AIP Conference Proceedings|year = 2016|last1 = England|first1 = R. J.|last2= Noble |first2= R. J. |last3= Fahimian |first3= B. |last4= Loo |first4= B. |last5= Abel |first5= E. |last6= Hanuka |first6= Adi |last7= Schachter |first7= L. |doi-access= free }}</ref> Using femtosecond duration laser pulses, an electron accelerating gradient 0.69 GeV/m was recorded for dielectric laser accelerators.<ref>{{Cite journal|last1=England|first1=R. Joel|last2=Byer|first2=Robert L.|last3=Soong|first3=Ken|last4=Peralta|first4=Edgar A.|last5=Makasyuk|first5=Igor V.|last6=Hanuka|first6=Adi|last7=Cowan|first7=Benjamin M.|last8=Wu|first8=Ziran|last9=Wootton|first9=Kent P.|date=2016-06-15|title=Demonstration of acceleration of relativistic electrons at a dielectric microstructure using femtosecond laser pulses|journal=Optics Letters|language=EN|volume=41|issue=12|pages=2696–2699|doi=10.1364/OL.41.002696|pmid=27304266|issn=1539-4794|bibcode=2016OptL...41.2696W|osti=1313076}}</ref> Higher gradients of the order of {{val|1|to|6|u=GeV/m}} are anticipated after further optimizations.<ref>{{Cite journal|date=2018-04-21|title=Operation regimes of a dielectric laser accelerator|journal=Nuclear Instruments and Methods in Physics Research Section A |language=en|volume=888|pages=147–152|doi=10.1016/j.nima.2018.01.060|issn=0168-9002|last1=Hanuka|first1=Adi|last2=Schächter|first2=Levi|bibcode=2018NIMPA.888..147H}}</ref> |
|||
===Advanced Accelerator Concepts=== |
|||
Advanced Accelerator Concepts encompasses methods of beam acceleration with gradients beyond state of the art in operational facilities. This includes diagnostics methods, timing technology, special needs for injectors, beam matching, beam dynamics and development of adequate simulations. Workshops dedicated to this subject are being held in the US (alternating locations) and in Europe, mostly on Isola d'[[Elba]]. |
|||
The series of Advanced Accelerator Concepts Workshops, held in the US,<ref>{{cite web | url=https://www.bnl.gov/atf/aac04.htm | title=Accelerator Concepts Workshop }}</ref> started as an international series in 1982.<ref>{{cite web | url=https://www.aac2022.org/aac-history/ | title=AAC22 - AAC History | date=4 January 2016 }}</ref> |
|||
The European Advanced Accelerator Concepts Workshop series started in 2019.<ref>{{cite web | url=http://www.lnf.infn.it/conference/EAAC2013/ | title=Eaac2013 }}</ref> |
|||
Topics related to Advanced Accelerator Concepts: |
|||
* Laser [[Plasma Acceleration]] of electrons and positrons |
|||
* Laser and High-Gradient Structure-Based Acceleration |
|||
* Beam-Driven Acceleration |
|||
* Laser-Plasma Acceleration of Ions |
|||
* Beam Sources such as [[electron gun]], Monitoring, and Control. See [[Accelerator physics]] |
|||
* [[Computer]] [[simulation]] for [[Accelerator Physics]] |
|||
* [[Laser]] technology for particle acceleration |
|||
* [[Electromagnetic radiation]] Generation |
|||
* [[Muon collider]] |
|||
According to the [[Inverse scattering problem]], any mechanism by which a particle produces radiation (where [[kinetic energy]] of the particle is transferred to the [[electromagnetic field]]), can be inverted such that the same radiation mechanism leads to the acceleration of the particle (energy of the radiation field is transferred to kinetic energy of the particle). The opposite is also true, any acceleration mechanism can be inverted to deposit the energy of the particle into a decelerating field, like in a [[kinetic energy recovery system]]. This is the idea enabling an [[energy recovery linac]]. |
|||
This principle, which is also behind the plasma or dielectric wakefield accelerrators, led to a few other interesting developments in advanced accelerator concepts: |
|||
* [[Cherenkov radiation]] led to inverse Cherenkov radiation accelerator.<ref>W. D. Kimura, G. H. Kim, R. D. Romea, et al, Laser Acceleration of Relativistic Electrons Using the Inverse Cherenkov Effect, Phys. Rev. Lett. ‘’’74’’’, 546 – Published 23 January 1995</ref> |
|||
* [[Free-electron laser]] led to the Inverse Free-electron laser accelerator.<ref>W. D. Kimura, A. van Steenbergen, M. Babzien, et al, First Staging of Two Laser Accelerators, Physical Review Letters '''86''' no. 18, 4041 (2001)</ref> |
|||
* A [[laser]] can also be inverted to produce acceleration of electrons.<ref>Samer Banna, Valery Berezovsky, and Levi Schächter, Experimental Observation of Direct Particle Acceleration by Stimulated Emission of Radiation, Phys. Rev. Lett. ‘’’97’’’, 134801 – Published 28 September 2006</ref> |
|||
=== Black hole production and public safety concerns === |
|||
==Higher energies== |
|||
{{See also|Safety of high energy particle collision experiments}} |
|||
At present the highest energy accelerators are all circular colliders, but it is likely that limits have been reached in respect of compensating for synchrotron radiation losses for electron accelerators, and the next generation will probably be linear accelerators 10 times the current length. An example of such a next generation electron accelerator is the 40 km long [[International Linear Collider]], due to be constructed between 2015-2020. |
|||
In the future, the possibility of a black hole production at the highest energy accelerators may arise if certain predictions of [[superstring theory]] are accurate.<ref>{{cite web |date=July 2004 |title=An Interview with Dr. Steve Giddings |url=http://www.esi-opics.com/blackholes/interviews/SteveGiddings.html |work=[[Essential Science Indicators|ESI Special Topics]] |publisher=[[Thomson Reuters]] |access-date=2014-08-02 |archive-date=2017-10-16 |archive-url=https://web.archive.org/web/20171016230038/http://www.esi-opics.com/blackholes/interviews/SteveGiddings.html |url-status=dead }}</ref><ref>{{cite journal |last1=Chamblin |first1=A. |last2=Nayak |first2=G. C. |year=2002 |title=Black hole production at the CERN LHC: String balls and black holes from pp and lead-lead collisions |journal=[[Physical Review D]] |volume=66 |issue=9 |pages=091901|doi=10.1103/PhysRevD.66.091901|arxiv=hep-ph/0206060 |bibcode=2002PhRvD..66i1901C|s2cid=119445499 }}</ref> This and other possibilities have led to [[Safety of particle collisions at the Large Hadron Collider|public safety concerns]] that have been widely reported in connection with the [[LHC]], which began operation in 2008. The various possible dangerous scenarios have been assessed as presenting "no conceivable danger" in the latest risk assessment produced by the LHC Safety Assessment Group.<ref name="LSAGreport">{{cite journal |last=Ellis |first=J. [[Large Hadron Collider|LHC Safety Assessment Group]] |date=5 September 2008|title=Review of the Safety of LHC Collisions |url=http://lsag.web.cern.ch/lsag/LSAG-Report.pdf |journal=[[Journal of Physics G]]|volume=35 |issue=11 |pages=115004 |arxiv=0806.3414 |doi=10.1088/0954-3899/35/11/115004 |id=[http://cdsweb.cern.ch/record/1111112?ln=fr CERN record]|bibcode=2008JPhG...35k5004E|s2cid=53370175 |display-authors=etal}}</ref> If black holes are produced, it is theoretically predicted that such small black holes should evaporate extremely quickly via [[Bekenstein–Hawking radiation]], but which is as yet experimentally unconfirmed. If colliders can produce black holes, [[cosmic ray]]s (and particularly [[ultra-high-energy cosmic ray]]s, UHECRs) must have been producing them for eons, but they have yet to harm anybody.<ref name="jaffe">{{cite journal |last1=Jaffe |first1=R. |last2=Busza |first2=W. |last3=Sandweiss |first3=J. |last4=Wilczek |first4=F. |year=2000 |title=Review of Speculative "Disaster Scenarios" at RHIC|journal=[[Reviews of Modern Physics]] |volume=72 |issue=4 |pages=1125–1140 |arxiv=hep-ph/9910333 |doi=10.1103/RevModPhys.72.1125|bibcode=2000RvMP...72.1125J|s2cid=444580 }}</ref> It has been argued that to conserve energy and momentum, any black holes created in a collision between an UHECR and local matter would necessarily be produced moving at relativistic speed with respect to the Earth, and should escape into space, as their accretion and growth rate should be very slow, while black holes produced in colliders (with components of equal mass) would have some chance of having a velocity less than Earth escape velocity, 11.2 km per sec, and would be liable to capture and subsequent growth. Yet even on such scenarios the collisions of UHECRs with white dwarfs and neutron stars would lead to their rapid destruction, but these bodies are observed to be common astronomical objects. Thus if stable micro black holes should be produced, they must grow far too slowly to cause any noticeable macroscopic effects within the natural lifetime of the solar system.<ref name="LSAGreport" /> |
|||
As of 2005, it is believed that [[plasma acceleration|plasma wakefield acceleration]] in the form of electron-beam 'afterburners' and standalone laser pulsers will provide dramatic increases in efficiency within two to three decades. In plasma wakefield accelerators, the beam cavity is filled with a plasma (rather than vacuum). A short pulse of electrons or laser light either constitutes or immediately trails the particles that are being accelerated. The pulse disrupts the plasma, causing the charged particles in the plasma to integrate into and move toward the rear of the bunch of particles that are being accelerated. This process transfers energy to the particle bunch, accelerating it further, and continues as long as the pulse is coherent.<ref>Matthew Early Wright (April 2005). "[http://www.symmetrymag.org/cms/?pid=1000091 Riding the Plasma Wave of the Future]". ''Symmetry: Dimensions of Particle Physics (Fermilab/SLAC)'', p. 12.</ref> |
|||
== Accelerator operator == |
|||
Energy gradients as steep as 200 GeV/m have been achieved over millimeter-scale distances using laser pulsers<ref>Briezman, et al. "[http://peaches.ph.utexas.edu/ifs/ifsreports/Self-focused762.pdf Self-Focused Particle Beam Drivers for Plasma Wakefield Accelerators]." (PDF) Retrieved 13 May 2005.</ref> and gradients approaching 1 GeV/m are being produced on the multi-centimeter-scale with electron-beam systems, in contrast to a limit of about 0.1 GeV/m for radio-frequency acceleration alone. Existing electron accelerators such as [[SLAC]] could use electron-beam afterburners to greatly increase the energy of their particle beams, at the cost of beam intensity. Electron systems in general can provide tightly collimated, reliable beams; laser systems may offer more power and compactness. Thus, plasma wakefield accelerators could be used — if technical issues can be resolved — to both increase the maximum energy of the largest accelerators and to bring high energies into university laboratories and medical centres. |
|||
The use of advanced technologies such as superconductivity, cryogenics, and high powered radiofrequency amplifiers, as well as the presence of ionizing radiation, pose challenges for the safe operation of accelerator facilities.<ref>{{cite book|last=Otto|first=Thomas|url=http://link.springer.com/10.1007/978-3-030-57031-6|title=Safety for Particle Accelerators|publisher=Springer International Publishing|year=2021|isbn=978-3-030-57030-9|series=Particle Acceleration and Detection|location=Cham|language=en|doi=10.1007/978-3-030-57031-6|bibcode=2021spa..book.....O |s2cid=234329600}}</ref><ref>{{cite book |last1=Cossairt |first1=J. Donald |url=https://www.taylorfrancis.com/books/9780429958496 |title=Accelerator Radiation Physics for Personnel and Environmental Protection |last2=Quinn |first2=Matthew |year=2019 |isbn=978-0-429-49163-4 |edition=1 |location=Boca Raton, FL |publisher=CRC Press, Taylor & Francis Group, [2019]|language=en|doi=10.1201/9780429491634|s2cid=189160205 }}</ref> An '''accelerator operator''' controls the operation of a particle accelerator, adjusts operating parameters such as [[aspect ratio]], current intensity, and position on target. They communicate with and assist accelerator maintenance personnel to ensure readiness of support systems, such as [[vacuum]], [[magnets]], magnetic and radiofrequency [[power supplies]] and controls, and cooling systems. Additionally, the accelerator operator maintains a record of accelerator related events. |
|||
== See also == |
|||
===Black hole production and public safety concerns=== |
|||
{{Portal|Physics}} |
|||
{{related|[[Large Hadron Collider#Safety of particle_collisions|Safety of particle collisions]]}} |
|||
* [[Accelerator physics]] |
|||
In the future, the possibility of [[black hole]] production at the highest energy accelerators may arise if certain predictions of [[superstring theory]] are accurate.<ref>An Interview with Dr. Steve Giddings http://www.esi-topics.com/blackholes/interviews/SteveGiddings.html</ref><ref>Phys. Rev. D 66, 091901 (2002) http://prola.aps.org/abstract/PRD/v66/i9/e091901</ref> |
|||
* [[Atom smasher (disambiguation)]] |
|||
This and other exotic possibilities have led to [[Safety of particle collisions at the Large Hadron Collider|public safety concerns]] that have been widely reported in connection with the LHC, which began operation in 2008. |
|||
* [[Compact Linear Collider]] |
|||
The various possible dangerous scenarios have been assessed as presenting "no conceivable danger" in the latest risk assessment produced by the LHC Safety Assessment Group.<ref>{{cite web|url=http://lsag.web.cern.ch/lsag/LSAG-Report.pdf|title=Review of the Safety of LHC Collisions: LHC Safety Assessment Group|format=PDF}}</ref> |
|||
* [[Dielectric wall accelerator]] |
|||
If they are produced, it is proposed that black holes would evaporate extremely quickly via the unconfirmed theory of [[Bekenstein-Hawking radiation]]. |
|||
* [[Future Circular Collider]] |
|||
If colliders can produce black holes, [[cosmic ray]]s (and particularly [[ultra-high-energy cosmic ray]]s, UHECRs) must have been producing them for eons, but they have yet to harm us.<ref name="jaffe">{{cite web|url=http://arxiv.org/abs/hep-ph/9910333|title=R. Jaffe ''et al.'', Rev. Mod. Phys. '''72''', 1125–1140 (2000).}}</ref> |
|||
* [[International Linear Collider]] |
|||
It has been argued that to conserve energy and momentum, any black holes created in a collision between an UHECR and local matter would necessarily be produced moving at relativistic speed with respect to the Earth, and should escape into space, as their accretion and growth rate should be very slow, while black holes produced in colliders (with components of equal mass) would have some chance of having a velocity less than Earth escape velocity, 11.2 km per sec, and would be liable to capture and subsequent growth. |
|||
* [[KALI (electron accelerator)|KALI]] |
|||
Yet even on such scenarios the collisions of UHECRs with white dwarfs and neutron stars would lead to their rapid destruction, but these bodies are observed to be common astronomical objects. |
|||
* [[Linear particle accelerator]] |
|||
Thus if stable micro black holes should be produced, they must grow far too slowly to cause any noticeable macroscopic effects within the natural lifetime of the solar system.<ref name="LSAGreport">Ellis J, Giudice G, Mangano ML, Tkachev I, Wiedemann U (LHC Safety Assessment Group) (5 September 2008). "[http://www.iop.org/EJ/article/0954-3899/35/11/115004/g8_11_115004.pdf?request-id=1973667e-34da-47a4-b75a-08624558a81b Review of the Safety of LHC Collisions]". ''''[[Journal of Physics G: Nuclear and Particle Physics]]''. 35, 115004 (18pp). [[doi:10.1088/0954-3899/35/11/115004]]. [http://cdsweb.cern.ch/record/1111112?ln=fr CERN record]. [[arXiv:0806.3414]].</ref> |
|||
* [[List of accelerators in particle physics]] |
|||
* [[Momentum compaction]] |
|||
* [[Nuclear transmutation]] |
|||
* [[Rolf Widerøe]] |
|||
* [[Superconducting Super Collider]] |
|||
==References== |
== References == |
||
{{reflist|colwidth=30em}} |
|||
{{Reflist}} |
|||
==External links== |
== External links == |
||
{{Commons category|Particle accelerators}} |
|||
{{refbegin}} |
|||
* [http://www2.slac.stanford.edu/vvc/accelerator.html What are particle accelerators used for?] |
* [http://www2.slac.stanford.edu/vvc/accelerator.html What are particle accelerators used for?] |
||
* Stanley Humphries (1999) [http://www.fieldp.com/cpa.html Principles of Charged Particle Acceleration] |
* Stanley Humphries (1999) [http://www.fieldp.com/cpa.html Principles of Charged Particle Acceleration] |
||
* [http://www-elsa.physik.uni-bonn.de/accelerator_list.html Particle Accelerators around the world] |
* [http://www-elsa.physik.uni-bonn.de/accelerator_list.html Particle Accelerators around the world] |
||
* Wolfgang K. H. Panofsky: [http://www.slac.stanford.edu/pubs/beamline/27/1/27-1-panofsky.pdf The Evolution of Particle Accelerators & Colliders], ([[PDF]]), Stanford, 1997 |
* Wolfgang K. H. Panofsky: [http://www.slac.stanford.edu/pubs/beamline/27/1/27-1-panofsky.pdf The Evolution of Particle Accelerators & Colliders], ([[PDF]]), Stanford, 1997 |
||
* P.J. Bryant, [https://cds.cern.ch/record/261062/files/p1_2.pdf A Brief History and Review of Accelerators] (PDF), [[CERN]], 1994. |
|||
* [http://bc1.lbl.gov/CBP_pages/educational/WoB/index2.htm UCB/LBL Beam Physics site] |
|||
* {{cite book |last=Heilbron |first=J.L. |author-link=J.L. Heilbron |author2=Robert W. Seidel |title=''Lawrence and His Laboratory: A History of the Lawrence Berkeley Laboratory'' |publisher=[[University of California Press]] |year=1989 |location=Berkeley |url=https://archive.org/details/lawrencehislabor00heil |isbn=978-0-520-06426-3}} |
|||
* P.J. Bryant, [http://documents.cern.ch/archive/cernrep/1994/94-01/p1.pdf A Brief History and Review of Accelerators] (PDF), [[CERN]], 1994. |
|||
* David Kestenbaum, [https://www.npr.org/templates/story/story.php?storyId=9433495 Massive Particle Accelerator Revving Up] NPR's Morning Edition article on 9 April 2007 |
|||
* {{cite book| last = Heilbron| first = J.L.| authorlink = J.L. Heilbron| coauthors = Robert W. Seidel | title = ''Lawrence and His Laboratory: A History of the Lawrence Berkeley Laboratory'' | publisher = [[University of California Press]] | date = 1989 | location = Berkeley | url = http://ark.cdlib.org/ark:/13030/ft5s200764/ | id = ISBN 0-520-06426-7 }} |
|||
* {{cite book |editor=Ragnar Hellborg |title=Electrostatic Accelerators: Fundamentals and Applications |publisher=Springer |year=2005 |url=https://books.google.com/books?id=tc6CEuIV1jEC&q=electrostatic+accelerator+book&pg=PA51 |isbn=978-3-540-23983-3}} |
|||
* David Kestenbaum, [http://www.npr.org/templates/story/story.php?storyId=9433495 Massive Particle Accelerator Revving Up] NPR's Morning Edition article on 9 April 2007 |
|||
* [http://alsos.wlu.edu/qsearch.aspx?browse=science/Particle+Accelerators Annotated bibliography for particle accelerators from the Alsos Digital Library for Nuclear Issues] {{Webarchive|url=https://web.archive.org/web/20101007075456/http://alsos.wlu.edu/qsearch.aspx?browse=science%2FParticle+Accelerators |date=2010-10-07 }} |
|||
* {{cite book|editor=Ragnar Hellborg (ed.)|title=Electrostatic Accelerators: Fundamentals and Applications|publisher=Springer|year=2005|url=http://books.google.com/books?id=tc6CEuIV1jEC&pg=PA51&lpg=PA51&dq=electrostatic+accelerator+book&source=web&ots=Qa0DbmiZJt&sig=bLoYaz_VUpBr7-Wv4lk_fLBnUo4#PPP1,M1}} |
|||
* [http://www.accelerators-for-society.org Accelerators-for-Society.org], to know more about applications of accelerators for Research and Development, energy and environment, health and medicine, industry, material characterization. |
|||
* [http://old-www.ansto.gov.au/natfac/antares.html ANTARES Tandem Accelerator] |
|||
{{refend}} |
|||
* [http://www2.tandar.cnea.gov.ar TANDAR Tandem Accelerator (Argentina)] |
|||
* [http://www.phy.ornl.gov/hribf/accelerator/tandemweb/ The Tandem Electrostatic Accelerator at ORNL] |
|||
* [http://www.niell.org/research.html Fred's World of Science] |
|||
* [http://www.rtftechnologies.org/physics/linac.htm RTFTechnologies.org Electrostatic Particle Accelerator] |
|||
* [http://thecyclotronkids.org A group of high school students building their own particle accelerator] |
|||
* [http://en.wikipedia.org/enwiki/w/index.php?title=Particle_accelerator&action=edit§ion=20 Fermilab 'closing in' on the God particle] |
|||
{{Levels of technological manipulation of matter}} |
|||
{{Radiation}} |
{{Radiation}} |
||
{{Authority control}} |
|||
<!-- {{Link GA|es}} This code affects the iw info --> |
|||
[[Category:Particle accelerators]] |
|||
[[Category:Types of magnets]] |
|||
[[Category:American inventions]] |
|||
{{DEFAULTSORT:Particle Accelerator}} |
|||
[[af:Partikelversneller]] |
|||
[[Category:Particle accelerators| ]] |
|||
[[ar:معجل جسيمات]] |
|||
[[bg:Ускорител на частици]] |
|||
[[ca:Accelerador de partícules]] |
|||
[[cs:Urychlovač částic]] |
|||
[[da:Partikelaccelerator]] |
|||
[[de:Teilchenbeschleuniger]] |
|||
[[el:Επιταχυντής σωματιδίων]] |
|||
[[es:Acelerador de partículas]] |
|||
[[eo:Partikla akcelilo]] |
|||
[[fa:شتابدهنده ذرهای]] |
|||
[[fr:Accélérateur de particules]] |
|||
[[gl:Acelerador de partículas]] |
|||
[[ko:입자 가속기]] |
|||
[[hi:कण त्वरक]] |
|||
[[hr:Akcelerator čestica]] |
|||
[[id:Pemercepat partikel]] |
|||
[[is:Eindahraðall]] |
|||
[[it:Acceleratore di particelle]] |
|||
[[he:מאיץ חלקיקים]] |
|||
[[kn:ಕಣ ಉತ್ಕರ್ಷಕ]] |
|||
[[la:Particularum acceleratorium]] |
|||
[[lt:Dalelių greitintuvas]] |
|||
[[hu:Részecskegyorsító]] |
|||
[[ml:കണികാത്വരണി]] |
|||
[[nl:Deeltjesversneller]] |
|||
[[ja:加速器]] |
|||
[[no:Partikkelakselerator]] |
|||
[[nn:Partikkelakselerator]] |
|||
[[pl:Akcelerator cząstek]] |
|||
[[pt:Acelerador de partículas]] |
|||
[[ro:Accelerator de particule]] |
|||
[[ru:Ускоритель заряженных частиц]] |
|||
[[simple:Particle accelerator]] |
|||
[[sk:Urýchľovač častíc]] |
|||
[[sl:Pospeševalnik]] |
|||
[[sr:Акцелератор]] |
|||
[[fi:Hiukkaskiihdytin]] |
|||
[[sv:Partikelaccelerator]] |
|||
[[tr:Parçacık hızlandırıcı]] |
|||
[[tk:Induksion tizlendiriji]] |
|||
[[uk:Прискорювач]] |
|||
[[ur:ذراتی مسرع]] |
|||
[[vi:Máy gia tốc hạt]] |
|||
[[zh:粒子加速器]] |
Latest revision as of 03:18, 13 December 2024
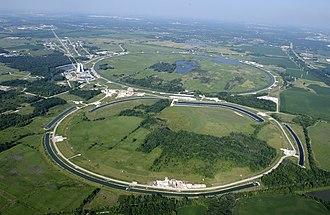

A particle accelerator is a machine that uses electromagnetic fields to propel charged particles to very high speeds and energies to contain them in well-defined beams.[1][2] Small accelerators are used for fundamental research in particle physics. Accelerators are also used as synchrotron light sources for the study of condensed matter physics. Smaller particle accelerators are used in a wide variety of applications, including particle therapy for oncological purposes, radioisotope production for medical diagnostics, ion implanters for the manufacturing of semiconductors, and accelerator mass spectrometers for measurements of rare isotopes such as radiocarbon.
Large accelerators include the Relativistic Heavy Ion Collider at Brookhaven National Laboratory in New York, and the largest accelerator, the Large Hadron Collider near Geneva, Switzerland, operated by CERN. It is a collider accelerator, which can accelerate two beams of protons to an energy of 6.5 TeV and cause them to collide head-on, creating center-of-mass energies of 13 TeV. There are more than 30,000 accelerators in operation around the world.[3][4]
There are two basic classes of accelerators: electrostatic and electrodynamic (or electromagnetic) accelerators.[5] Electrostatic particle accelerators use static electric fields to accelerate particles. The most common types are the Cockcroft–Walton generator and the Van de Graaff generator. A small-scale example of this class is the cathode-ray tube in an ordinary old television set. The achievable kinetic energy for particles in these devices is determined by the accelerating voltage, which is limited by electrical breakdown. Electrodynamic or electromagnetic accelerators, on the other hand, use changing electromagnetic fields (either magnetic induction or oscillating radio frequency fields) to accelerate particles. Since in these types the particles can pass through the same accelerating field multiple times, the output energy is not limited by the strength of the accelerating field. This class, which was first developed in the 1920s, is the basis for most modern large-scale accelerators.
Rolf Widerøe, Gustav Ising, Leó Szilárd, Max Steenbeck, and Ernest Lawrence are considered pioneers of this field, having conceived and built the first operational linear particle accelerator,[6] the betatron, as well as the cyclotron. Because the target of the particle beams of early accelerators was usually the atoms of a piece of matter, with the goal being to create collisions with their nuclei in order to investigate nuclear structure, accelerators were commonly referred to as atom smashers in the 20th century.[7] The term persists despite the fact that many modern accelerators create collisions between two subatomic particles, rather than a particle and an atomic nucleus.[8][9][10]
Uses
[edit]
Beams of high-energy particles are useful for fundamental and applied research in the sciences and also in many technical and industrial fields unrelated to fundamental research.[11] There are approximately 30,000 accelerators worldwide; of these, only about 1% are research machines with energies above 1 GeV, while about 44% are for radiotherapy, 41% for ion implantation, 9% for industrial processing and research, and 4% for biomedical and other low-energy research.[12]
Particle physics
[edit]For the most basic inquiries into the dynamics and structure of matter, space, and time, physicists seek the simplest kinds of interactions at the highest possible energies. These typically entail particle energies of many GeV, and interactions of the simplest kinds of particles: leptons (e.g. electrons and positrons) and quarks for the matter, or photons and gluons for the field quanta. Since isolated quarks are experimentally unavailable due to color confinement, the simplest available experiments involve the interactions of, first, leptons with each other, and second, of leptons with nucleons, which are composed of quarks and gluons. To study the collisions of quarks with each other, scientists resort to collisions of nucleons, which at high energy may be usefully considered as essentially 2-body interactions of the quarks and gluons of which they are composed. This elementary particle physicists tend to use machines creating beams of electrons, positrons, protons, and antiprotons, interacting with each other or with the simplest nuclei (e.g., hydrogen or deuterium) at the highest possible energies, generally hundreds of GeV or more.
The largest and highest-energy particle accelerator used for elementary particle physics is the Large Hadron Collider (LHC) at CERN, operating since 2009.[13]
Nuclear physics and isotope production
[edit]Nuclear physicists and cosmologists may use beams of bare atomic nuclei, stripped of electrons, to investigate the structure, interactions, and properties of the nuclei themselves, and of condensed matter at extremely high temperatures and densities, such as might have occurred in the first moments of the Big Bang. These investigations often involve collisions of heavy nuclei – of atoms like iron or gold – at energies of several GeV per nucleon. The largest such particle accelerator is the Relativistic Heavy Ion Collider (RHIC) at Brookhaven National Laboratory.
Particle accelerators can also produce proton beams, which can produce proton-rich medical or research isotopes as opposed to the neutron-rich ones made in fission reactors; however, recent work has shown how to make 99Mo, usually made in reactors, by accelerating isotopes of hydrogen,[14] although this method still requires a reactor to produce tritium. An example of this type of machine is LANSCE at Los Alamos National Laboratory.
Synchrotron radiation
[edit]Electrons propagating through a magnetic field emit very bright and coherent photon beams via synchrotron radiation. It has numerous uses in the study of atomic structure, chemistry, condensed matter physics, biology, and technology. A large number of synchrotron light sources exist worldwide. Examples in the U.S. are SSRL at SLAC National Accelerator Laboratory, APS at Argonne National Laboratory, ALS at Lawrence Berkeley National Laboratory, and NSLS-II at Brookhaven National Laboratory. In Europe, there are MAX IV in Lund, Sweden, BESSY in Berlin, Germany, Diamond in Oxfordshire, UK, ESRF in Grenoble, France, the latter has been used to extract detailed 3-dimensional images of insects trapped in amber.[15]
Free-electron lasers (FELs) are a special class of light sources based on synchrotron radiation that provides shorter pulses with higher temporal coherence. A specially designed FEL is the most brilliant source of x-rays in the observable universe.[16] The most prominent examples are the LCLS in the U.S. and European XFEL in Germany. More attention is being drawn towards soft x-ray lasers, which together with pulse shortening opens up new methods for attosecond science.[17] Apart from x-rays, FELs are used to emit terahertz light, e.g. FELIX in Nijmegen, Netherlands, TELBE in Dresden, Germany and NovoFEL in Novosibirsk, Russia.
Thus there is a great demand for electron accelerators of moderate (GeV) energy, high intensity and high beam quality to drive light sources.
Low-energy machines and particle therapy
[edit]Everyday examples of particle accelerators are cathode ray tubes found in television sets and X-ray generators. These low-energy accelerators use a single pair of electrodes with a DC voltage of a few thousand volts between them. In an X-ray generator, the target itself is one of the electrodes. A low-energy particle accelerator called an ion implanter is used in the manufacture of integrated circuits.
At lower energies, beams of accelerated nuclei are also used in medicine as particle therapy, for the treatment of cancer.
DC accelerator types capable of accelerating particles to speeds sufficient to cause nuclear reactions are Cockcroft–Walton generators or voltage multipliers, which convert AC to high voltage DC, or Van de Graaff generators that use static electricity carried by belts.
Radiation sterilization of medical devices
[edit]Electron beam processing is commonly used for sterilization. Electron beams are an on-off technology that provide a much higher dose rate than gamma or X-rays emitted by radioisotopes like cobalt-60 (60Co) or caesium-137 (137Cs). Due to the higher dose rate, less exposure time is required and polymer degradation is reduced. Because electrons carry a charge, electron beams are less penetrating than both gamma and X-rays.[18]
Electrostatic particle accelerators
[edit]

Historically, the first accelerators used simple technology of a single static high voltage to accelerate charged particles. The charged particle was accelerated through an evacuated tube with an electrode at either end, with the static potential across it. Since the particle passed only once through the potential difference, the output energy was limited to the accelerating voltage of the machine. While this method is still extremely popular today, with the electrostatic accelerators greatly out-numbering any other type, they are more suited to lower energy studies owing to the practical voltage limit of about 1 MV for air insulated machines, or 30 MV when the accelerator is operated in a tank of pressurized gas with high dielectric strength, such as sulfur hexafluoride. In a tandem accelerator the potential is used twice to accelerate the particles, by reversing the charge of the particles while they are inside the terminal. This is possible with the acceleration of atomic nuclei by using anions (negatively charged ions), and then passing the beam through a thin foil to strip electrons off the anions inside the high voltage terminal, converting them to cations (positively charged ions), which are accelerated again as they leave the terminal.
The two main types of electrostatic accelerator are the Cockcroft–Walton accelerator, which uses a diode-capacitor voltage multiplier to produce high voltage, and the Van de Graaff accelerator, which uses a moving fabric belt to carry charge to the high voltage electrode. Although electrostatic accelerators accelerate particles along a straight line, the term linear accelerator is more often used for accelerators that employ oscillating rather than static electric fields.
Electrodynamic (electromagnetic) particle accelerators
[edit]Due to the high voltage ceiling imposed by electrical discharge, in order to accelerate particles to higher energies, techniques involving dynamic fields rather than static fields are used. Electrodynamic acceleration can arise from either of two mechanisms: non-resonant magnetic induction, or resonant circuits or cavities excited by oscillating radio frequency (RF) fields.[19] Electrodynamic accelerators can be linear, with particles accelerating in a straight line, or circular, using magnetic fields to bend particles in a roughly circular orbit.
Magnetic induction accelerators
[edit]Magnetic induction accelerators accelerate particles by induction from an increasing magnetic field, as if the particles were the secondary winding in a transformer. The increasing magnetic field creates a circulating electric field which can be configured to accelerate the particles. Induction accelerators can be either linear or circular.
Linear induction accelerators
[edit]Linear induction accelerators utilize ferrite-loaded, non-resonant induction cavities. Each cavity can be thought of as two large washer-shaped disks connected by an outer cylindrical tube. Between the disks is a ferrite toroid. A voltage pulse applied between the two disks causes an increasing magnetic field which inductively couples power into the charged particle beam.[20]
The linear induction accelerator was invented by Christofilos in the 1960s.[21] Linear induction accelerators are capable of accelerating very high beam currents (>1000 A) in a single short pulse. They have been used to generate X-rays for flash radiography (e.g. DARHT at LANL), and have been considered as particle injectors for magnetic confinement fusion and as drivers for free electron lasers.
Betatrons
[edit]The Betatron is a circular magnetic induction accelerator, invented by Donald Kerst in 1940 for accelerating electrons. The concept originates ultimately from Norwegian-German scientist Rolf Widerøe.[22][23] These machines, like synchrotrons, use a donut-shaped ring magnet (see below) with a cyclically increasing B field, but accelerate the particles by induction from the increasing magnetic field, as if they were the secondary winding in a transformer, due to the changing magnetic flux through the orbit.[24][25]
Achieving constant orbital radius while supplying the proper accelerating electric field requires that the magnetic flux linking the orbit be somewhat independent of the magnetic field on the orbit, bending the particles into a constant radius curve. These machines have in practice been limited by the large radiative losses suffered by the electrons moving at nearly the speed of light in a relatively small radius orbit.
Linear accelerators
[edit]
In a linear particle accelerator (linac), particles are accelerated in a straight line with a target of interest at one end. They are often used to provide an initial low-energy kick to particles before they are injected into circular accelerators. The longest linac in the world is the Stanford Linear Accelerator, SLAC, which is 3 km (1.9 mi) long. SLAC was originally an electron–positron collider but is now a X-ray Free-electron laser.
Linear high-energy accelerators use a linear array of plates (or drift tubes) to which an alternating high-energy field is applied. As the particles approach a plate they are accelerated towards it by an opposite polarity charge applied to the plate. As they pass through a hole in the plate, the polarity is switched so that the plate now repels them and they are now accelerated by it towards the next plate. Normally a stream of "bunches" of particles are accelerated, so a carefully controlled AC voltage is applied to each plate to continuously repeat this process for each bunch.
As the particles approach the speed of light the switching rate of the electric fields becomes so high that they operate at radio frequencies, and so microwave cavities are used in higher energy machines instead of simple plates.
Linear accelerators are also widely used in medicine, for radiotherapy and radiosurgery. Medical grade linacs accelerate electrons using a klystron and a complex bending magnet arrangement which produces a beam of energy 6–30 MeV. The electrons can be used directly or they can be collided with a target to produce a beam of X-rays. The reliability, flexibility and accuracy of the radiation beam produced has largely supplanted the older use of cobalt-60 therapy as a treatment tool.
Circular or cyclic RF accelerators
[edit]In the circular accelerator, particles move in a circle until they reach enough energy. The particle track is typically bent into a circle using electromagnets. The advantage of circular accelerators over linear accelerators (linacs) is that the ring topology allows continuous acceleration, as the particle can transit indefinitely. Another advantage is that a circular accelerator is smaller than a linear accelerator of comparable power (i.e. a linac would have to be extremely long to have the equivalent power of a circular accelerator).
Depending on the energy and the particle being accelerated, circular accelerators suffer a disadvantage in that the particles emit synchrotron radiation. When any charged particle is accelerated, it emits electromagnetic radiation and secondary emissions. As a particle traveling in a circle is always accelerating towards the center of the circle, it continuously radiates towards the tangent of the circle. This radiation is called synchrotron light and depends highly on the mass of the accelerating particle. For this reason, many high energy electron accelerators are linacs. Certain accelerators (synchrotrons) are however built specially for producing synchrotron light (X-rays).
Since the special theory of relativity requires that matter always travels slower than the speed of light in vacuum, in high-energy accelerators, as the energy increases the particle speed approaches the speed of light as a limit, but never attains it. Therefore, particle physicists do not generally think in terms of speed, but rather in terms of a particle's energy or momentum, usually measured in electron volts (eV). An important principle for circular accelerators, and particle beams in general, is that the curvature of the particle trajectory is proportional to the particle charge and to the magnetic field, but inversely proportional to the (typically relativistic) momentum.
Cyclotrons
[edit]
The earliest operational circular accelerators were cyclotrons, invented in 1929 by Ernest Lawrence at the University of California, Berkeley. Cyclotrons have a single pair of hollow D-shaped plates to accelerate the particles and a single large dipole magnet to bend their path into a circular orbit. It is a characteristic property of charged particles in a uniform and constant magnetic field B that they orbit with a constant period, at a frequency called the cyclotron frequency, so long as their speed is small compared to the speed of light c. This means that the accelerating D's of a cyclotron can be driven at a constant frequency by a RF accelerating power source, as the beam spirals outwards continuously. The particles are injected in the center of the magnet and are extracted at the outer edge at their maximum energy.
Cyclotrons reach an energy limit because of relativistic effects whereby the particles effectively become more massive, so that their cyclotron frequency drops out of sync with the accelerating RF. Therefore, simple cyclotrons can accelerate protons only to an energy of around 15 million electron volts (15 MeV, corresponding to a speed of roughly 10% of c), because the protons get out of phase with the driving electric field. If accelerated further, the beam would continue to spiral outward to a larger radius but the particles would no longer gain enough speed to complete the larger circle in step with the accelerating RF. To accommodate relativistic effects the magnetic field needs to be increased to higher radii as is done in isochronous cyclotrons. An example of an isochronous cyclotron is the PSI Ring cyclotron in Switzerland, which provides protons at the energy of 590 MeV which corresponds to roughly 80% of the speed of light. The advantage of such a cyclotron is the maximum achievable extracted proton current which is currently 2.2 mA. The energy and current correspond to 1.3 MW beam power which is the highest of any accelerator currently existing.
Synchrocyclotrons and isochronous cyclotrons
[edit]
A classic cyclotron can be modified to increase its energy limit. The historically first approach was the synchrocyclotron, which accelerates the particles in bunches. It uses a constant magnetic field , but reduces the accelerating field's frequency so as to keep the particles in step as they spiral outward, matching their mass-dependent cyclotron resonance frequency. This approach suffers from low average beam intensity due to the bunching, and again from the need for a huge magnet of large radius and constant field over the larger orbit demanded by high energy.
The second approach to the problem of accelerating relativistic particles is the isochronous cyclotron. In such a structure, the accelerating field's frequency (and the cyclotron resonance frequency) is kept constant for all energies by shaping the magnet poles so to increase magnetic field with radius. Thus, all particles get accelerated in isochronous time intervals. Higher energy particles travel a shorter distance in each orbit than they would in a classical cyclotron, thus remaining in phase with the accelerating field. The advantage of the isochronous cyclotron is that it can deliver continuous beams of higher average intensity, which is useful for some applications. The main disadvantages are the size and cost of the large magnet needed, and the difficulty in achieving the high magnetic field values required at the outer edge of the structure.
Synchrocyclotrons have not been built since the isochronous cyclotron was developed.
Synchrotrons
[edit]
To reach still higher energies, with relativistic mass approaching or exceeding the rest mass of the particles (for protons, billions of electron volts or GeV), it is necessary to use a synchrotron. This is an accelerator in which the particles are accelerated in a ring of constant radius. An immediate advantage over cyclotrons is that the magnetic field need only be present over the actual region of the particle orbits, which is much narrower than that of the ring. (The largest cyclotron built in the US had a 184-inch-diameter (4.7 m) magnet pole, whereas the diameter of synchrotrons such as the LEP and LHC is nearly 10 km. The aperture of the two beams of the LHC is of the order of a centimeter.) The LHC contains 16 RF cavities, 1232 superconducting dipole magnets for beam steering, and 24 quadrupoles for beam focusing.[26] Even at this size, the LHC is limited by its ability to steer the particles without them going adrift. This limit is theorized to occur at 14 TeV.[27]
However, since the particle momentum increases during acceleration, it is necessary to turn up the magnetic field B in proportion to maintain constant curvature of the orbit. In consequence, synchrotrons cannot accelerate particles continuously, as cyclotrons can, but must operate cyclically, supplying particles in bunches, which are delivered to a target or an external beam in beam "spills" typically every few seconds.
Since high energy synchrotrons do most of their work on particles that are already traveling at nearly the speed of light c, the time to complete one orbit of the ring is nearly constant, as is the frequency of the RF cavity resonators used to drive the acceleration.
In modern synchrotrons, the beam aperture is small and the magnetic field does not cover the entire area of the particle orbit as it does for a cyclotron, so several necessary functions can be separated. Instead of one huge magnet, one has a line of hundreds of bending magnets, enclosing (or enclosed by) vacuum connecting pipes. The design of synchrotrons was revolutionized in the early 1950s with the discovery of the strong focusing concept.[28][29][30] The focusing of the beam is handled independently by specialized quadrupole magnets, while the acceleration itself is accomplished in separate RF sections, rather similar to short linear accelerators.[31] Also, there is no necessity that cyclic machines be circular, but rather the beam pipe may have straight sections between magnets where beams may collide, be cooled, etc. This has developed into an entire separate subject, called "beam physics" or "beam optics".[32]
More complex modern synchrotrons such as the Tevatron, LEP, and LHC may deliver the particle bunches into storage rings of magnets with a constant magnetic field, where they can continue to orbit for long periods for experimentation or further acceleration. The highest-energy machines such as the Tevatron and LHC are actually accelerator complexes, with a cascade of specialized elements in series, including linear accelerators for initial beam creation, one or more low energy synchrotrons to reach intermediate energy, storage rings where beams can be accumulated or "cooled" (reducing the magnet aperture required and permitting tighter focusing; see beam cooling), and a last large ring for final acceleration and experimentation.

Electron synchrotrons
[edit]Circular electron accelerators fell somewhat out of favor for particle physics around the time that SLAC's linear particle accelerator was constructed, because their synchrotron losses were considered economically prohibitive and because their beam intensity was lower than for the unpulsed linear machines. The Cornell Electron Synchrotron, built at low cost in the late 1970s, was the first in a series of high-energy circular electron accelerators built for fundamental particle physics, the last being LEP, built at CERN, which was used from 1989 until 2000.
A large number of electron synchrotrons have been built in the past two decades, as part of synchrotron light sources that emit ultraviolet light and X rays; see below.
Synchrotron radiation sources
[edit]Some circular accelerators have been built to deliberately generate radiation (called synchrotron light) as X-rays also called synchrotron radiation, for example the Diamond Light Source which has been built at the Rutherford Appleton Laboratory in England or the Advanced Photon Source at Argonne National Laboratory in Illinois, USA. High-energy X-rays are useful for X-ray spectroscopy of proteins or X-ray absorption fine structure (XAFS), for example.
Synchrotron radiation is more powerfully emitted by lighter particles, so these accelerators are invariably electron accelerators. Synchrotron radiation allows for better imaging as researched and developed at SLAC's SPEAR.
Fixed-field alternating gradient accelerators
[edit]Fixed-Field Alternating Gradient accelerators (FFA)s, in which a magnetic field which is fixed in time, but with a radial variation to achieve strong focusing, allows the beam to be accelerated with a high repetition rate but in a much smaller radial spread than in the cyclotron case. Isochronous FFAs, like isochronous cyclotrons, achieve continuous beam operation, but without the need for a huge dipole bending magnet covering the entire radius of the orbits. Some new developments in FFAs are covered in.[33]
Rhodotron
[edit]
A Rhodotron is an industrial electron accelerator first proposed in 1987 by J. Pottier of the French Atomic Energy Agency (CEA),[34] manufactured by Belgian company Ion Beam Applications. It accelerates electrons by recirculating them across the diameter of a cylinder-shaped radiofrequency cavity. A Rhodotron has an electron gun, which emits an electron beam that is attracted to a pillar in the center of the cavity. The pillar has holes the electrons can pass through. The electron beam passes through the pillar via one of these holes and then travels through a hole in the wall of the cavity, and meets a bending magnet, the beam is then bent and sent back into the cavity, to another hole in the pillar, the electrons then again go across the pillar and pass though another part of the wall of the cavity and into another bending magnet, and so on, gradually increasing the energy of the beam until it is allowed to exit the cavity for use. The cylinder and pillar may be lined with copper on the inside.[35][36][37]
History
[edit]Ernest Lawrence's first cyclotron was a mere 4 inches (100 mm) in diameter. Later, in 1939, he built a machine with a 60-inch diameter pole face, and planned one with a 184-inch diameter in 1942, which was, however, taken over for World War II-related work connected with uranium isotope separation; after the war it continued in service for research and medicine over many years.
The first large proton synchrotron was the Cosmotron at Brookhaven National Laboratory, which accelerated protons to about 3 GeV (1953–1968). The Bevatron at Berkeley, completed in 1954, was specifically designed to accelerate protons to enough energy to create antiprotons, and verify the particle–antiparticle symmetry of nature, then only theorized. The Alternating Gradient Synchrotron (AGS) at Brookhaven (1960–) was the first large synchrotron with alternating gradient, "strong focusing" magnets, which greatly reduced the required aperture of the beam, and correspondingly the size and cost of the bending magnets. The Proton Synchrotron, built at CERN (1959–), was the first major European particle accelerator and generally similar to the AGS.
The Stanford Linear Accelerator, SLAC, became operational in 1966, accelerating electrons to 30 GeV in a 3 km long waveguide, buried in a tunnel and powered by hundreds of large klystrons. It is still the largest linear accelerator in existence, and has been upgraded with the addition of storage rings and an electron-positron collider facility. It is also an X-ray and UV synchrotron photon source.
The Fermilab Tevatron has a ring with a beam path of 4 miles (6.4 km). It has received several upgrades, and has functioned as a proton-antiproton collider until it was shut down due to budget cuts on September 30, 2011. The largest circular accelerator ever built was the LEP synchrotron at CERN with a circumference 26.6 kilometers, which was an electron/positron collider. It achieved an energy of 209 GeV before it was dismantled in 2000 so that the tunnel could be used for the Large Hadron Collider (LHC). The LHC is a proton collider, and currently the world's largest and highest-energy accelerator, achieving 6.5 TeV energy per beam (13 TeV in total).
The aborted Superconducting Super Collider (SSC) in Texas would have had a circumference of 87 km. Construction was started in 1991, but abandoned in 1993. Very large circular accelerators are invariably built in tunnels a few metres wide to minimize the disruption and cost of building such a structure on the surface, and to provide shielding against intense secondary radiations that occur, which are extremely penetrating at high energies.
Current accelerators such as the Spallation Neutron Source, incorporate superconducting cryomodules. The Relativistic Heavy Ion Collider, and Large Hadron Collider also make use of superconducting magnets and RF cavity resonators to accelerate particles.
Targets
[edit]The output of a particle accelerator can generally be directed towards multiple lines of experiments, one at a given time, by means of a deviating electromagnet. This makes it possible to operate multiple experiments without needing to move things around or shutting down the entire accelerator beam. Except for synchrotron radiation sources, the purpose of an accelerator is to generate high-energy particles for interaction with matter.
This is usually a fixed target, such as the phosphor coating on the back of the screen in the case of a television tube; a piece of uranium in an accelerator designed as a neutron source; or a tungsten target for an X-ray generator. In a linac, the target is simply fitted to the end of the accelerator. The particle track in a cyclotron is a spiral outwards from the centre of the circular machine, so the accelerated particles emerge from a fixed point as for a linear accelerator.
For synchrotrons, the situation is more complex. Particles are accelerated to the desired energy. Then, a fast acting dipole magnet is used to switch the particles out of the circular synchrotron tube and towards the target.
A variation commonly used for particle physics research is a collider, also called a storage ring collider. Two circular synchrotrons are built in close proximity – usually on top of each other and using the same magnets (which are then of more complicated design to accommodate both beam tubes). Bunches of particles travel in opposite directions around the two accelerators and collide at intersections between them. This can increase the energy enormously; whereas in a fixed-target experiment the energy available to produce new particles is proportional to the square root of the beam energy, in a collider the available energy is linear.
Detectors NA
[edit]The detectors gather clues about the particles including their speed and charge. Using these, the scientists can actually work on the particle. The process of detection is very complex it requires strong electromagnets and accelerators to generate enough usable information.
![]() | This section is empty. You can help by adding to it. (September 2024) |
Higher energies
[edit]At present the highest energy accelerators are all circular colliders, but both hadron accelerators and electron accelerators are running into limits. Higher energy hadron and ion cyclic accelerators will require accelerator tunnels of larger physical size due to the increased beam rigidity.
For cyclic electron accelerators, a limit on practical bend radius is placed by synchrotron radiation losses and the next generation will probably be linear accelerators 10 times the current length. An example of such a next generation electron accelerator is the proposed 40 km long International Linear Collider.
It is believed that plasma wakefield acceleration in the form of electron-beam "afterburners" and standalone laser pulsers might be able to provide dramatic increases in efficiency over RF accelerators within two to three decades. In plasma wakefield accelerators, the beam cavity is filled with a plasma (rather than vacuum). A short pulse of electrons or laser light either constitutes or immediately precedes the particles that are being accelerated. The pulse disrupts the plasma, causing the charged particles in the plasma to integrate into and move toward the rear of the bunch of particles that are being accelerated. This process transfers energy to the particle bunch, accelerating it further, and continues as long as the pulse is coherent.[38]
Energy gradients as steep as 200 GeV/m have been achieved over millimeter-scale distances using laser pulsers[39] and gradients approaching 1 GeV/m are being produced on the multi-centimeter-scale with electron-beam systems, in contrast to a limit of about 0.1 GeV/m for radio-frequency acceleration alone. Existing electron accelerators such as SLAC could use electron-beam afterburners to greatly increase the energy of their particle beams, at the cost of beam intensity. Electron systems in general can provide tightly collimated, reliable beams; laser systems may offer more power and compactness. Thus, plasma wakefield accelerators could be used – if technical issues can be resolved – to both increase the maximum energy of the largest accelerators and to bring high energies into university laboratories and medical centres.
Higher than 0.25 GeV/m gradients have been achieved by a dielectric laser accelerator,[40] which may present another viable approach to building compact high-energy accelerators.[41] Using femtosecond duration laser pulses, an electron accelerating gradient 0.69 GeV/m was recorded for dielectric laser accelerators.[42] Higher gradients of the order of 1 to 6 GeV/m are anticipated after further optimizations.[43]
Advanced Accelerator Concepts
[edit]Advanced Accelerator Concepts encompasses methods of beam acceleration with gradients beyond state of the art in operational facilities. This includes diagnostics methods, timing technology, special needs for injectors, beam matching, beam dynamics and development of adequate simulations. Workshops dedicated to this subject are being held in the US (alternating locations) and in Europe, mostly on Isola d'Elba. The series of Advanced Accelerator Concepts Workshops, held in the US,[44] started as an international series in 1982.[45] The European Advanced Accelerator Concepts Workshop series started in 2019.[46] Topics related to Advanced Accelerator Concepts:
- Laser Plasma Acceleration of electrons and positrons
- Laser and High-Gradient Structure-Based Acceleration
- Beam-Driven Acceleration
- Laser-Plasma Acceleration of Ions
- Beam Sources such as electron gun, Monitoring, and Control. See Accelerator physics
- Computer simulation for Accelerator Physics
- Laser technology for particle acceleration
- Electromagnetic radiation Generation
- Muon collider
According to the Inverse scattering problem, any mechanism by which a particle produces radiation (where kinetic energy of the particle is transferred to the electromagnetic field), can be inverted such that the same radiation mechanism leads to the acceleration of the particle (energy of the radiation field is transferred to kinetic energy of the particle). The opposite is also true, any acceleration mechanism can be inverted to deposit the energy of the particle into a decelerating field, like in a kinetic energy recovery system. This is the idea enabling an energy recovery linac. This principle, which is also behind the plasma or dielectric wakefield accelerrators, led to a few other interesting developments in advanced accelerator concepts:
- Cherenkov radiation led to inverse Cherenkov radiation accelerator.[47]
- Free-electron laser led to the Inverse Free-electron laser accelerator.[48]
- A laser can also be inverted to produce acceleration of electrons.[49]
Black hole production and public safety concerns
[edit]In the future, the possibility of a black hole production at the highest energy accelerators may arise if certain predictions of superstring theory are accurate.[50][51] This and other possibilities have led to public safety concerns that have been widely reported in connection with the LHC, which began operation in 2008. The various possible dangerous scenarios have been assessed as presenting "no conceivable danger" in the latest risk assessment produced by the LHC Safety Assessment Group.[52] If black holes are produced, it is theoretically predicted that such small black holes should evaporate extremely quickly via Bekenstein–Hawking radiation, but which is as yet experimentally unconfirmed. If colliders can produce black holes, cosmic rays (and particularly ultra-high-energy cosmic rays, UHECRs) must have been producing them for eons, but they have yet to harm anybody.[53] It has been argued that to conserve energy and momentum, any black holes created in a collision between an UHECR and local matter would necessarily be produced moving at relativistic speed with respect to the Earth, and should escape into space, as their accretion and growth rate should be very slow, while black holes produced in colliders (with components of equal mass) would have some chance of having a velocity less than Earth escape velocity, 11.2 km per sec, and would be liable to capture and subsequent growth. Yet even on such scenarios the collisions of UHECRs with white dwarfs and neutron stars would lead to their rapid destruction, but these bodies are observed to be common astronomical objects. Thus if stable micro black holes should be produced, they must grow far too slowly to cause any noticeable macroscopic effects within the natural lifetime of the solar system.[52]
Accelerator operator
[edit]The use of advanced technologies such as superconductivity, cryogenics, and high powered radiofrequency amplifiers, as well as the presence of ionizing radiation, pose challenges for the safe operation of accelerator facilities.[54][55] An accelerator operator controls the operation of a particle accelerator, adjusts operating parameters such as aspect ratio, current intensity, and position on target. They communicate with and assist accelerator maintenance personnel to ensure readiness of support systems, such as vacuum, magnets, magnetic and radiofrequency power supplies and controls, and cooling systems. Additionally, the accelerator operator maintains a record of accelerator related events.
See also
[edit]- Accelerator physics
- Atom smasher (disambiguation)
- Compact Linear Collider
- Dielectric wall accelerator
- Future Circular Collider
- International Linear Collider
- KALI
- Linear particle accelerator
- List of accelerators in particle physics
- Momentum compaction
- Nuclear transmutation
- Rolf Widerøe
- Superconducting Super Collider
References
[edit]- ^ Chao, Alexander W; Chou, Weiren (2008). Reviews of Accelerator Science and Technology: Volume 1. Singapore: World Scientific. Bibcode:2008rast.book.....C. doi:10.1142/7037. ISBN 978-981-283-520-8.
- ^ Livingston, M. S.; Blewett, J. (1969). Particle Accelerators. New York: McGraw-Hill. ISBN 978-1-114-44384-6.
- ^ "More background on accelerators". www.iaea.org. 2016-10-12. Retrieved 2023-11-10.
- ^ Witman, Sarah (15 April 2014). "Ten things you might not know about particle accelerators". Symmetry Magazine. Fermi National Accelerator Laboratory. Retrieved 21 April 2014.
- ^ Humphries, Stanley (1986). Principles of Charged Particle Acceleration. Wiley-Interscience. p. 4. ISBN 978-0471878780.
- ^ Sessler, Andrew; Wilson, Edmund (2014). Engines of Discovery: A Century of Particle Accelerators Revised and Expanded Edition. World Scientific. Bibcode:2014edcp.book.....S. doi:10.1142/8552. ISBN 978-981-4417-18-1.
- ^ "six Million Volt Atom Smasher Creates New Elements". Popular Mechanics: 580. April 1935.
- ^ Higgins, A. G. (December 18, 2009). "Atom Smasher Preparing 2010 New Science Restart". U.S. News & World Report.
- ^ Cho, A. (June 2, 2006). "Aging Atom Smasher Runs All Out in Race for Most Coveted Particle". Science. 312 (5778): 1302–1303. doi:10.1126/science.312.5778.1302. PMID 16741091. S2CID 7016336.
- ^ "Atom smasher". American Heritage Science Dictionary. Houghton Mifflin Harcourt. 2005. p. 49. ISBN 978-0-618-45504-1.
- ^ Möller, Sören (2020). Accelerator Technology: Applications in Science, Medicine, and Industry. Particle Acceleration and Detection. Cham: Springer International Publishing. doi:10.1007/978-3-030-62308-1. ISBN 978-3-030-62307-4. S2CID 229610872.
- ^ Feder, T. (2010). "Accelerator school travels university circuit" (PDF). Physics Today. 63 (2): 20–22. Bibcode:2010PhT....63b..20F. doi:10.1063/1.3326981.
- ^ "Two circulating beams bring first collisions in the LHC" (Press release). CERN Press Office. November 23, 2009. Retrieved 2009-11-23.
- ^ Nagai, Y.; Hatsukawa, Y. (2009). "Production of 99Mo for Nuclear Medicine by 100Mo(n,2n)99Mo". Journal of the Physical Society of Japan. 78 (3): 033201. Bibcode:2009JPSJ...78c3201N. doi:10.1143/JPSJ.78.033201.
- ^ Amos, J. (April 1, 2008). "Secret 'dino bugs' revealed". BBC News. Retrieved 2008-09-11.
- ^ Ullrich, Joachim; Rudenko, Artem; Moshammer, Robert (2012-04-04). "Free-Electron Lasers: New Avenues in Molecular Physics and Photochemistry". Annual Review of Physical Chemistry. 63 (1): 635–660. Bibcode:2012ARPC...63..635U. doi:10.1146/annurev-physchem-032511-143720. ISSN 0066-426X. PMID 22404584.
- ^ Mak, Alan; Shamuilov, Georgii; Salén, Peter; Dunning, David; Hebling, János; Kida, Yuichiro; Kinjo, Ryota; McNeil, Brian W J; Tanaka, Takashi; Thompson, Neil; Tibai, Zoltán (2019-02-01). "Attosecond single-cycle undulator light: a review". Reports on Progress in Physics. 82 (2): 025901. Bibcode:2019RPPh...82b5901M. doi:10.1088/1361-6633/aafa35. ISSN 0034-4885. PMID 30572315. S2CID 58632996.
- ^ "2019 Midwest Medical Device Sterilization Workshop: Summary Report" (PDF). United States Department of Energy. November 2019.
- ^ Humphries, Stanley (1986). Principles of Charged Particle Acceleration. Wiley-Interscience. p. 6. ISBN 978-0471878780.
- ^ Humphries, Stanley (1986). "Linear Induction Accelerators". Principles of Charged Particle Acceleration. Wiley-Interscience. pp. 283–325. ISBN 978-0471878780.
- ^ Christofilos, N.C.; et al. (1963). "High-current linear induction accelerator for electrons". Proceedings, 4th International Conference on High-Energy Accelerators (HEACC63) (PDF). pp. 1482–1488.
- ^ Sørheim, Aashild (5 November 2019). Obsessed by a Dream: The Physicist Rolf Widerøe – a Giant in the History of Accelerators. Springer Biographies. Cham: Springer International Publishing. doi:10.1007/978-3-030-26338-6. ISBN 978-3-030-26337-9. S2CID 211929538.
- ^ Pedro Waloschek (ed.): The Infancy of Particle Accelerators: Life and Work of Rolf Wideröe, Vieweg, 1994
- ^ Chao, A. W.; Mess, K. H.; Tigner, M.; et al., eds. (2013). Handbook of Accelerator Physics and Engineering (2nd ed.). World Scientific. doi:10.1142/8543. ISBN 978-981-4417-17-4. S2CID 108427390.
- ^ Humphries, Stanley (1986). "Betatrons". Principles of Charged Particle Acceleration. Wiley-Interscience. p. 326ff. ISBN 978-0471878780.
- ^ ["Pulling together: Superconducting electromagnets" CERN; https://home.cern/science/engineering/pulling-together-superconducting-electromagnets Archived 2020-04-23 at the Wayback Machine]
- ^ ["Restarting the LHC: Why 13 TeV?" CERN; https://home.cern/science/engineering/restarting-lhc-why-13-tev Archived 2018-10-07 at the Wayback Machine]
- ^ Courant, E. D.; Livingston, M. S.; Snyder, H. S. (1952). "The Strong-Focusing Synchrotron—A New High Energy Accelerator". Physical Review. 88 (5): 1190–1196. Bibcode:1952PhRv...88.1190C. doi:10.1103/PhysRev.88.1190. hdl:2027/mdp.39015086454124.
- ^ Blewett, J. P. (1952). "Radial Focusing in the Linear Accelerator". Physical Review. 88 (5): 1197–1199. Bibcode:1952PhRv...88.1197B. doi:10.1103/PhysRev.88.1197.
- ^ "The Alternating Gradient Concept". Brookhaven National Laboratory. Archived from the original on 2013-04-02. Retrieved 2009-04-29.
- ^ Efimov, S.P.; Korenev, I.L.; Yudin, L.A. (1990). "Resonances of electron beam focused by a helical quadrupole magnetic field". Radiophysics and Quantum Electronics. 33 (1): 88–95. doi:10.1007/BF01037825. S2CID 123706289.
- ^ "World of Beams Homepage". Lawrence Berkeley National Laboratory. Archived from the original on 2005-03-02. Retrieved 2009-04-29.
- ^ Clery, D. (2010). "The Next Big Beam?". Science. 327 (5962): 142–144. Bibcode:2010Sci...327..142C. doi:10.1126/science.327.5962.142. PMID 20056871.
- ^ Chao, Alexander Wu; Mess, Karl Hubert (December 31, 2013). Handbook of Accelerator Physics and Engineering. World Scientific. ISBN 978-981-4415-85-9 – via Google Books.
- ^ Reviews of Accelerator Science and Technology: Accelerator Applications in Industry and the Environment. World Scientific. 20 February 2012. ISBN 978-981-4383-98-1.
- ^ Industrial Accelerators and Their Applications. World Scientific. 27 June 2012. ISBN 978-981-4434-61-4.
- ^ Jongen, Y.; Abs, M.; Capdevila, J.M.; Defrise, D.; Genin, F.; Nguyen, A. (1994). "The Rhodotron, a new high-energy, high-power, CW electron accelerator". Nuclear Instruments and Methods in Physics Research Section B. 89 (1–4): 60–64. Bibcode:1994NIMPB..89...60J. doi:10.1016/0168-583X(94)95146-2.
- ^ Wright, M. E. (April 2005). "Riding the Plasma Wave of the Future". Symmetry Magazine. 2 (3): 12. Archived from the original on 2006-10-02. Retrieved 2005-11-10.
- ^ Briezman, B. N.; et al. (1997). "Self-Focused Particle Beam Drivers for Plasma Wakefield Accelerators" (PDF). AIP Conference Proceedings. 396: 75–88. Bibcode:1997AIPC..396...75B. doi:10.1063/1.52975. Archived from the original (PDF) on 2005-05-23. Retrieved 2005-05-13.
- ^ Peralta, E. A.; et al. (2013). "Demonstration of electron acceleration in a laser-driven dielectric microstructure". Nature. 503 (7474): 91–94. Bibcode:2013Natur.503...91P. doi:10.1038/nature12664. PMID 24077116. S2CID 4467824.
- ^ England, R. J.; Noble, R. J.; Fahimian, B.; Loo, B.; Abel, E.; Hanuka, Adi; Schachter, L. (2016). "Conceptual layout for a wafer-scale dielectric laser accelerator". AIP Conference Proceedings. 1777: 060002. doi:10.1063/1.4965631.
- ^ England, R. Joel; Byer, Robert L.; Soong, Ken; Peralta, Edgar A.; Makasyuk, Igor V.; Hanuka, Adi; Cowan, Benjamin M.; Wu, Ziran; Wootton, Kent P. (2016-06-15). "Demonstration of acceleration of relativistic electrons at a dielectric microstructure using femtosecond laser pulses". Optics Letters. 41 (12): 2696–2699. Bibcode:2016OptL...41.2696W. doi:10.1364/OL.41.002696. ISSN 1539-4794. OSTI 1313076. PMID 27304266.
- ^ Hanuka, Adi; Schächter, Levi (2018-04-21). "Operation regimes of a dielectric laser accelerator". Nuclear Instruments and Methods in Physics Research Section A. 888: 147–152. Bibcode:2018NIMPA.888..147H. doi:10.1016/j.nima.2018.01.060. ISSN 0168-9002.
- ^ "Accelerator Concepts Workshop".
- ^ "AAC22 - AAC History". 4 January 2016.
- ^ "Eaac2013".
- ^ W. D. Kimura, G. H. Kim, R. D. Romea, et al, Laser Acceleration of Relativistic Electrons Using the Inverse Cherenkov Effect, Phys. Rev. Lett. ‘’’74’’’, 546 – Published 23 January 1995
- ^ W. D. Kimura, A. van Steenbergen, M. Babzien, et al, First Staging of Two Laser Accelerators, Physical Review Letters 86 no. 18, 4041 (2001)
- ^ Samer Banna, Valery Berezovsky, and Levi Schächter, Experimental Observation of Direct Particle Acceleration by Stimulated Emission of Radiation, Phys. Rev. Lett. ‘’’97’’’, 134801 – Published 28 September 2006
- ^ "An Interview with Dr. Steve Giddings". ESI Special Topics. Thomson Reuters. July 2004. Archived from the original on 2017-10-16. Retrieved 2014-08-02.
- ^ Chamblin, A.; Nayak, G. C. (2002). "Black hole production at the CERN LHC: String balls and black holes from pp and lead-lead collisions". Physical Review D. 66 (9): 091901. arXiv:hep-ph/0206060. Bibcode:2002PhRvD..66i1901C. doi:10.1103/PhysRevD.66.091901. S2CID 119445499.
- ^ a b Ellis, J. LHC Safety Assessment Group; et al. (5 September 2008). "Review of the Safety of LHC Collisions" (PDF). Journal of Physics G. 35 (11): 115004. arXiv:0806.3414. Bibcode:2008JPhG...35k5004E. doi:10.1088/0954-3899/35/11/115004. S2CID 53370175. CERN record.
- ^ Jaffe, R.; Busza, W.; Sandweiss, J.; Wilczek, F. (2000). "Review of Speculative "Disaster Scenarios" at RHIC". Reviews of Modern Physics. 72 (4): 1125–1140. arXiv:hep-ph/9910333. Bibcode:2000RvMP...72.1125J. doi:10.1103/RevModPhys.72.1125. S2CID 444580.
- ^ Otto, Thomas (2021). Safety for Particle Accelerators. Particle Acceleration and Detection. Cham: Springer International Publishing. Bibcode:2021spa..book.....O. doi:10.1007/978-3-030-57031-6. ISBN 978-3-030-57030-9. S2CID 234329600.
- ^ Cossairt, J. Donald; Quinn, Matthew (2019). Accelerator Radiation Physics for Personnel and Environmental Protection (1 ed.). Boca Raton, FL: CRC Press, Taylor & Francis Group, [2019]. doi:10.1201/9780429491634. ISBN 978-0-429-49163-4. S2CID 189160205.
External links
[edit]- What are particle accelerators used for?
- Stanley Humphries (1999) Principles of Charged Particle Acceleration
- Particle Accelerators around the world
- Wolfgang K. H. Panofsky: The Evolution of Particle Accelerators & Colliders, (PDF), Stanford, 1997
- P.J. Bryant, A Brief History and Review of Accelerators (PDF), CERN, 1994.
- Heilbron, J.L.; Robert W. Seidel (1989). Lawrence and His Laboratory: A History of the Lawrence Berkeley Laboratory. Berkeley: University of California Press. ISBN 978-0-520-06426-3.
- David Kestenbaum, Massive Particle Accelerator Revving Up NPR's Morning Edition article on 9 April 2007
- Ragnar Hellborg, ed. (2005). Electrostatic Accelerators: Fundamentals and Applications. Springer. ISBN 978-3-540-23983-3.
- Annotated bibliography for particle accelerators from the Alsos Digital Library for Nuclear Issues Archived 2010-10-07 at the Wayback Machine
- Accelerators-for-Society.org, to know more about applications of accelerators for Research and Development, energy and environment, health and medicine, industry, material characterization.