Mitochondrion: Difference between revisions
ClueBot NG (talk | contribs) m Reverting possible vandalism by 50.196.66.17 to version by Materialscientist. False positive? Report it. Thanks, ClueBot NG. (1994945) (Bot) |
No edit summary |
||
Line 3: | Line 3: | ||
The '''mitochondrion''' (plural '''mitochondria''') is a [[biological membrane|membrane]] [[organelle]] found in most [[eukaryotic]] [[cell (biology)|cells]] (the cells that make up [[plant]]s, [[animal]]s, [[fungi]], and many other forms of life).<ref name="mitosomes">{{cite journal |author=Henze K, Martin W |title=Evolutionary biology: essence of mitochondria |journal=Nature |volume=426 |issue=6963 |pages=127–8 |year=2003 |pmid=14614484 |doi=10.1038/426127a |last2=Martin |first2=William}}</ref> The word mitochondrion comes from the [[Greek language|Greek]] {{lang|el|''μίτος''}}, {{transl|el|''mitos''}}, i.e. "thread", and {{lang|el|''χονδρίον''}}, {{transl|el|''chondrion''}}, i.e. "granule".<ref name=OnlineEtDict>{{cite web|title=mitochondria|url=http://www.etymonline.com/index.php?term=mitochondria&allowed_in_frame=0|publisher=[[Online Etymology Dictionary]]}}</ref> |
The '''mitochondrion''' (plural '''mitochondria''') is a [[biological membrane|membrane]] [[organelle]] found in most [[eukaryotic]] [[cell (biology)|cells]] (the cells that make up [[plant]]s, [[animal]]s, [[fungi]], and many other forms of life).<ref name="mitosomes">{{cite journal |author=Henze K, Martin W |title=Evolutionary biology: essence of mitochondria |journal=Nature |volume=426 |issue=6963 |pages=127–8 |year=2003 |pmid=14614484 |doi=10.1038/426127a |last2=Martin |first2=William}}</ref> The word mitochondrion comes from the [[Greek language|Greek]] {{lang|el|''μίτος''}}, {{transl|el|''mitos''}}, i.e. "thread", and {{lang|el|''χονδρίον''}}, {{transl|el|''chondrion''}}, i.e. "granule".<ref name=OnlineEtDict>{{cite web|title=mitochondria|url=http://www.etymonline.com/index.php?term=mitochondria&allowed_in_frame=0|publisher=[[Online Etymology Dictionary]]}}</ref> |
||
baby: m-m |
|||
mother: mama? |
|||
baby: mitochondria is the power house of the cell! |
|||
:) |
|||
Mitochondria range from 0.5 to 1.0 micrometer ([[μm]]) in diameter. These structures are sometimes described as "cellular power plants" because they generate most of the cell's supply of [[adenosine triphosphate]] (ATP), used as a source of [[chemical energy]].<ref>{{cite book |last=Campbell |first=Neil A.|author2=Brad Williamson |author3=Robin J. Heyden |title=Biology: Exploring Life |publisher=Pearson Prentice Hall |year=2006 |location=Boston, Massachusetts|url=http://www.phschool.com/el_marketing.html |isbn=0-13-250882-6}}</ref> In addition to supplying cellular energy, mitochondria are involved in other tasks such as [[cell signaling|signaling]], [[cellular differentiation]], [[apoptosis|cell death]], as well as the control of the [[cell cycle]] and [[cell growth]].<ref>{{cite journal |author=McBride HM, Neuspiel M, Wasiak S |title=Mitochondria: more than just a powerhouse |journal=Curr. Biol. |volume=16 |issue=14 |pages=R551–60 |year=2006 |pmid=16860735 |doi=10.1016/j.cub.2006.06.054}}</ref> Mitochondria have been implicated in several human diseases, including [[mitochondrial disorders]]<ref>{{cite journal |author=Gardner A, Boles RG |title=Is a "Mitochondrial Psychiatry" in the Future? A Review |journal=Curr. Psychiatry Review |volume=1 |issue=3 |pages=255–271 |year=2005 |doi=10.2174/157340005774575064}}</ref> and [[cardiac]] dysfunction,<ref>{{Cite journal |author=Lesnefsky EJ, Moghaddas S, Tandler B, Kerner B, Hoppel CL |title=Mitochondrial dysfunction in cardiac disease: ischemia—reperfusion, aging, and heart failure |journal=[[Journal of Molecular and Cellular Cardiology]] |volume=33 |issue=6 |pages=1065–1089 |date=June 2001 |doi=10.1006/jmcc.2001.1378 |pmid=11444914}}</ref> and may play a role in the [[aging process]]. More recent research indicates that autism, especially severe autism, is correlated with mitochondrial defects.<ref name="biosciencetechnology.com">http://www.biosciencetechnology.com/news/2014/05/study-confirms-mitochondrial-deficits-children-autism</ref> |
Mitochondria range from 0.5 to 1.0 micrometer ([[μm]]) in diameter. These structures are sometimes described as "cellular power plants" because they generate most of the cell's supply of [[adenosine triphosphate]] (ATP), used as a source of [[chemical energy]].<ref>{{cite book |last=Campbell |first=Neil A.|author2=Brad Williamson |author3=Robin J. Heyden |title=Biology: Exploring Life |publisher=Pearson Prentice Hall |year=2006 |location=Boston, Massachusetts|url=http://www.phschool.com/el_marketing.html |isbn=0-13-250882-6}}</ref> In addition to supplying cellular energy, mitochondria are involved in other tasks such as [[cell signaling|signaling]], [[cellular differentiation]], [[apoptosis|cell death]], as well as the control of the [[cell cycle]] and [[cell growth]].<ref>{{cite journal |author=McBride HM, Neuspiel M, Wasiak S |title=Mitochondria: more than just a powerhouse |journal=Curr. Biol. |volume=16 |issue=14 |pages=R551–60 |year=2006 |pmid=16860735 |doi=10.1016/j.cub.2006.06.054}}</ref> Mitochondria have been implicated in several human diseases, including [[mitochondrial disorders]]<ref>{{cite journal |author=Gardner A, Boles RG |title=Is a "Mitochondrial Psychiatry" in the Future? A Review |journal=Curr. Psychiatry Review |volume=1 |issue=3 |pages=255–271 |year=2005 |doi=10.2174/157340005774575064}}</ref> and [[cardiac]] dysfunction,<ref>{{Cite journal |author=Lesnefsky EJ, Moghaddas S, Tandler B, Kerner B, Hoppel CL |title=Mitochondrial dysfunction in cardiac disease: ischemia—reperfusion, aging, and heart failure |journal=[[Journal of Molecular and Cellular Cardiology]] |volume=33 |issue=6 |pages=1065–1089 |date=June 2001 |doi=10.1006/jmcc.2001.1378 |pmid=11444914}}</ref> and may play a role in the [[aging process]]. More recent research indicates that autism, especially severe autism, is correlated with mitochondrial defects.<ref name="biosciencetechnology.com">http://www.biosciencetechnology.com/news/2014/05/study-confirms-mitochondrial-deficits-children-autism</ref> |
Revision as of 15:02, 17 October 2014

Cell biology | |
---|---|
![]() Components of a typical animal cell:
| |
![]() Components of a typical mitochondrion
3 Lamella
4 Mitochondrial DNA |
The mitochondrion (plural mitochondria) is a membrane organelle found in most eukaryotic cells (the cells that make up plants, animals, fungi, and many other forms of life).[1] The word mitochondrion comes from the Greek [μίτος] Error: {{Lang}}: text has italic markup (help), mitos, i.e. "thread", and [χονδρίον] Error: {{Lang}}: text has italic markup (help), chondrion, i.e. "granule".[2]
baby: m-m mother: mama? baby: mitochondria is the power house of the cell!
- )
Mitochondria range from 0.5 to 1.0 micrometer (μm) in diameter. These structures are sometimes described as "cellular power plants" because they generate most of the cell's supply of adenosine triphosphate (ATP), used as a source of chemical energy.[3] In addition to supplying cellular energy, mitochondria are involved in other tasks such as signaling, cellular differentiation, cell death, as well as the control of the cell cycle and cell growth.[4] Mitochondria have been implicated in several human diseases, including mitochondrial disorders[5] and cardiac dysfunction,[6] and may play a role in the aging process. More recent research indicates that autism, especially severe autism, is correlated with mitochondrial defects.[7]
Several characteristics make mitochondria unique. The number of mitochondria in a cell varies widely by organism and tissue type. Many cells have only a single mitochondrion, whereas others can contain several thousand mitochondria.[8][9] The organelle is composed of compartments that carry out specialized functions. These compartments or regions include the outer membrane, the intermembrane space, the inner membrane, and the cristae and matrix. Mitochondrial proteins vary depending on the tissue and the species. In humans, 615 distinct types of proteins have been identified from cardiac mitochondria,[10] whereas in rats, 940 proteins have been reported.[11] The mitochondrial proteome is thought to be dynamically regulated.[12] Although most of a cell's DNA is contained in the cell nucleus, the mitochondrion has its own independent genome. Further, its DNA shows substantial similarity to bacterial genomes.[13]
History
The first observations of intracellular structures that probably represent mitochondria were published in the 1840s.[14] Richard Altmann, in 1890, established them as cell organelles and called them "bioblasts".[15] The term "mitochondria" itself was coined by Carl Benda in 1898.[16][14] Leonor Michaelis discovered that Janus green can be used as a supravital stain for mitochondria in 1900. Friedrich Meves, in 1904, made the first recorded observation of mitochondria in plants (Nymphaea alba)[17] and in 1908, along with Claudius Regaud, suggested that they contain proteins and lipids. Benjamin F. Kingsbury, in 1912, first related them with cell respiration, but almost exclusively based on morphological observations.[14] In 1913 particles from extracts of guinea-pig liver were linked to respiration by Otto Heinrich Warburg, which he called "grana". Warburg and Heinrich Otto Wieland, who had also postulated a similar particle mechanism, disagreed on the chemical nature of the respiration. It was not until 1925 when David Keilin discovered cytochromes that the respiratory chain was described.[14]
In 1939, experiments using minced muscle cells demonstrated that one oxygen atom can form two adenosine triphosphate molecules, and, in 1941, the concept of phosphate bonds being a form of energy in cellular metabolism was developed by Fritz Albert Lipmann. In the following years, the mechanism behind cellular respiration was further elaborated, although its link to the mitochondria was not known.[14] The introduction of tissue fractionation by Albert Claude allowed mitochondria to be isolated from other cell fractions and biochemical analysis to be conducted on them alone. In 1946, he concluded that cytochrome oxidase and other enzymes responsible for the respiratory chain were isolated to the mitchondria. Over time, the fractionation method was tweaked, improving the quality of the mitochondria isolated, and other elements of cell respiration were determined to occur in the mitochondria.[14]
The first high-resolution micrographs appeared in 1952, replacing the Janus Green stains as the preferred way of visualising the mitochondria. This led to a more detailed analysis of the structure of the mitochondria, including confirmation that they were surrounded by a membrane. It also showed a second membrane inside the mitochondria that folded up in ridges dividing up the inner chamber and that the size and shape of the mitochondria varied from cell to cell.
The popular term "powerhouse of the cell" was coined by Philip Siekevitz in 1957.[18]
In 1967, it was discovered that mitochondria contained ribosomes. In 1968, methods were developed for mapping the mitochondrial genes, with the genetic and physical map of yeast mitochondria being completed in 1976.[14]
Structure
![]() | It has been suggested that Mitochondrial fission and Mitochondrial fusion be merged into this article. (Discuss) Proposed since May 2013. |

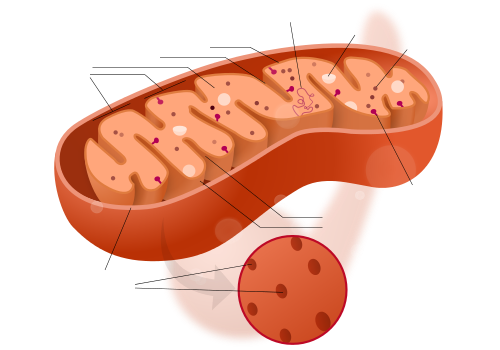

A mitochondrion contains outer and inner membranes composed of phospholipid bilayers and proteins.[8] The two membranes have different properties. Because of this double-membraned organization, there are five distinct parts to a mitochondrion. They are:
- the outer mitochondrial membrane,
- the intermembrane space (the space between the outer and inner membranes),
- the inner mitochondrial membrane,
- the cristae space (formed by infoldings of the inner membrane), and
- the matrix (space within the inner membrane).
Mitochondria stripped of their outer membrane are called mitoplasts.
Outer membrane
The outer mitochondrial membrane, which encloses the entire organelle, has a protein-to-phospholipid ratio similar to that of the eukaryotic plasma membrane (about 1:1 by weight). It contains large numbers of integral proteins called porins. These porins form channels that allow molecules 5000 daltons or less in molecular weight to freely diffuse from one side of the membrane to the other.[8] Larger proteins can enter the mitochondrion if a signaling sequence at their N-terminus binds to a large multisubunit protein called translocase of the outer membrane, which then actively moves them across the membrane.[20] Disruption of the outer membrane permits proteins in the intermembrane space to leak into the cytosol, leading to certain cell death.[21] The mitochondrial outer membrane can associate with the endoplasmic reticulum (ER) membrane, in a structure called MAM (mitochondria-associated ER-membrane). This is important in the ER-mitochondria calcium signaling and involved in the transfer of lipids between the ER and mitochondria.[22]
Intermembrane space
The intermembrane space is the space between the outer membrane and the inner membrane. It is also known as perimitochondrial space. Because the outer membrane is freely permeable to small molecules, the concentrations of small molecules such as ions and sugars in the intermembrane space is the same as the cytosol.[8] However, large proteins must have a specific signaling sequence to be transported across the outer membrane, so the protein composition of this space is different from the protein composition of the cytosol. One protein that is localized to the intermembrane space in this way is cytochrome c.[21]
Inner membrane
The inner mitochondrial membrane contains proteins with five types of functions:[8]
- Those that perform the redox reactions of oxidative phosphorylation
- ATP synthase, which generates ATP in the matrix
- Specific transport proteins that regulate metabolite passage into and out of the matrix
- Protein import machinery.
- Mitochondria fusion and fission protein.
It contains more than 151 different polypeptides, and has a very high protein-to-phospholipid ratio (more than 3:1 by weight, which is about 1 protein for 15 phospholipids). The inner membrane is home to around 1/5 of the total protein in a mitochondrion.[8] In addition, the inner membrane is rich in an unusual phospholipid, cardiolipin. This phospholipid was originally discovered in cow hearts in 1942, and is usually characteristic of mitochondrial and bacterial plasma membranes.[23] Cardiolipin contains four fatty acids rather than two, and may help to make the inner membrane impermeable.[8] Unlike the outer membrane, the inner membrane doesn't contain porins, and is highly impermeable to all molecules. Almost all ions and molecules require special membrane transporters to enter or exit the matrix. Proteins are ferried into the matrix via the translocase of the inner membrane (TIM) complex or via Oxa1.[20] In addition, there is a membrane potential across the inner membrane, formed by the action of the enzymes of the electron transport chain.
Cristae

The inner mitochondrial membrane is compartmentalized into numerous cristae, which expand the surface area of the inner mitochondrial membrane, enhancing its ability to produce ATP. For typical liver mitochondria, the area of the inner membrane is about five times as large as the outer membrane. This ratio is variable and mitochondria from cells that have a greater demand for ATP, such as muscle cells, contain even more cristae. These folds are studded with small round bodies known as F1 particles or oxysomes. These are not simple random folds but rather invaginations of the inner membrane, which can affect overall chemiosmotic function.[24]
One recent mathematical modeling study has suggested that the optical properties of the cristae in filamentous mitochondria may affect the generation and propagation of light within the tissue.[25]
Matrix
The matrix is the space enclosed by the inner membrane. It contains about 2/3 of the total protein in a mitochondrion.[8] The matrix is important in the production of ATP with the aid of the ATP synthase contained in the inner membrane. The matrix contains a highly concentrated mixture of hundreds of enzymes, special mitochondrial ribosomes, tRNA, and several copies of the mitochondrial DNA genome. Of the enzymes, the major functions include oxidation of pyruvate and fatty acids, and the citric acid cycle.[8]
Mitochondria have their own genetic material, and the machinery to manufacture their own RNAs and proteins (see: protein biosynthesis). A published human mitochondrial DNA sequence revealed 16,569 base pairs encoding 37 total genes: 22 tRNA, 2 rRNA, and 13 peptide genes.[26] The 13 mitochondrial peptides in humans are integrated into the inner mitochondrial membrane, along with proteins encoded by genes that reside in the host cell's nucleus.
Mitochondria-associated ER membrane (MAM)
The mitochondria-associated ER membrane (MAM) is another structural element that is increasingly recognized for its critical role in cellular physiology and homeostasis. Once considered a technical snag in cell fractionation techniques, the alleged ER vesicle contaminants that invariably appeared in the mitochondrial fraction have been re-identified as membranous structures derived from the MAM—the interface between mitochondria and the ER.[27] Physical coupling between these two organelles had previously been observed in electron micrographs and has more recently been probed with fluorescence microscopy.[27] Such studies estimate that at the MAM, which may comprise up to 20% of the mitochondrial outer membrane, the ER and mitochondria are separated by a mere 10–25 nm and held together by protein tethering complexes.[27][28][29]
Purified MAM from subcellular fractionation has shown to be enriched in enzymes involved in phospholipid exchange, in addition to channels associated with Ca2+ signaling.[27][29] These hints of a prominent role for the MAM in the regulation of cellular lipid stores and signal transduction have been borne out, with significant implications for mitochondrial-associated cellular phenomena, as discussed below. Not only has the MAM provided insight into the mechanistic basis underlying such physiological processes as intrinsic apoptosis and the propagation of calcium signaling, but it also favors a more refined view of the mitochondria. Though often seen as static, isolated 'powerhouses' hijacked for cellular metabolism through an ancient endosymbiotic event, the evolution of the MAM underscores the extent to which mitochondria have been integrated into overall cellular physiology, with intimate physical and functional coupling to the endomembrane system.
Phospholipid transfer
The MAM is enriched in enzymes involved in lipid biosynthesis, such as phosphatidylserine synthase on the ER face and phosphatidylserine decarboxylase on the mitochondrial face.[30][31] Because mitochondria are dynamic organelles constantly undergoing fission and fusion events, they require a constant and well-regulated supply of phospholipids for membrane integrity.[32][33] But mitochondria are not only a destination for the phospholipids they finish synthesis of; rather, this organelle also plays a role in inter-organelle trafficking of the intermediates and products of phospholipid biosynthetic pathways, ceramide and cholesterol metabolism, and glycosphingolipid anabolism.[31][33]
Such trafficking capacity depends on the MAM, which has been shown to facilitate transfer of lipid intermediates between organelles.[30] In contrast to the standard vesicular mechanism of lipid transfer, evidence indicates that the physical proximity of the ER and mitochondrial membranes at the MAM allows for lipid flipping between opposed bilayers.[33] Despite this unusual and seemingly energetically unfavorable mechanism, such transport does not require ATP.[33] Instead, in yeast, it has been shown to be dependent on a multiprotein tethering structure termed the ER-mitochondria encounter structure, or ERMES, although it remains unclear whether this structure directly mediates lipid transfer or is required to keep the membranes in sufficiently close proximity to lower the energy barrier for lipid flipping.[33][34]
The MAM may also be part of the secretory pathway, in addition to its role in intracellular lipid trafficking. In particular, the MAM appears to be an intermediate destination between the rough ER and the Golgi in the pathway that leads to very-low-density lipoprotein, or VLDL, assembly and secretion.[31][35] The MAM thus serves as a critical metabolic and trafficking hub in lipid metabolism.
Calcium signaling
A critical role for the ER in calcium signaling was acknowledged before such a role for the mitochondria was widely accepted, in part because the low affinity of Ca2+ channels localized to the outer mitochondrial membrane seemed to fly in the face of this organelle's purported responsiveness to changes in intracellular Ca2+ flux.[27] But the presence of the MAM resolves this apparent contradiction: the close physical association between the two organelles results in Ca2+ microdomains at contact points that facilitate efficient Ca2+ transmission from the ER to the mitochondria.[27] Transmission occurs in response to so-called "Ca2+ puffs" generated by spontaneous clustering and activation of IP3R, a canonical ER membrane Ca2+ channel.[27][28]
The fate of these puffs—in particular, whether they remain restricted to isolated locales or integrated into Ca2+ waves for propagation throughout the cell—is determined in large part by MAM dynamics. Although reuptake of Ca2+ by the ER (concomitant with its release) modulates the intensity of the puffs, thus insulating mitochondria to a certain degree from high Ca2+ exposure, the MAM often serves as a firewall that essentially buffers Ca2+ puffs by acting as a sink into which free ions released into the cytosol can be funneled.[27][36][37] This Ca2+ tunneling occurs through the low-affinity Ca2+ receptor VDAC1, which recently has been shown to be physically tethered to the IP3R clusters on the ER membrane and enriched at the MAM.[27][28][38] The ability of mitochondria to serve as a Ca2+ sink is a result of the electrochemical gradient generated during oxidative phosphorylation, which makes tunneling of the cation an exergonic process.[38]
But transmission of Ca2+ is not unidirectional; rather, it is a two-way street. The properties of the Ca2+ pump SERCA and the channel IP3R present on the ER membrane facilitate feedback regulation coordinated by MAM function. In particular, clearance of Ca2+ by the MAM allows for spatio-temporal patterning of Ca2+ signaling because Ca2+ alters IP3R activity in a biphasic manner.[27] SERCA is likewise affected by mitochondrial feedback: uptake of Ca2+ by the MAM stimulates ATP production, thus providing energy that enables SERCA to reload the ER with Ca2+ for continued Ca2+ efflux at the MAM.[36][38] Thus, the MAM is not a passive buffer for Ca2+ puffs; rather it helps modulate further Ca2+ signaling through feedback loops that affect ER dynamics.
Regulating ER release of Ca2+ at the MAM is especially critical because only a certain window of Ca2+ uptake sustains the mitochondria, and consequently the cell, at homeostasis. Sufficient intraorganelle Ca2+ signaling is required to stimulate metabolism by activating dehydrogenase enzymes critical to flux through the citric acid cycle.[39] However, once Ca2+ signaling in the mitochondria passes a certain threshold, it stimulates the intrinsic pathway of apoptosis in part by collapsing the mitochondrial membrane potential required for metabolism.[27] Studies examining the role of pro- and anti-apoptotic factors support this model; for example, the anti-apoptotic factor Bcl-2 has been shown to interact with IP3Rs to reduce Ca2+ filling of the ER, leading to reduced efflux at the MAM and preventing collapse of the mitochondrial membrane potential post-apoptotic stimuli.[27] Given the need for such fine regulation of Ca2+ signaling, it is perhaps unsurprising that dysregulated mitochondrial Ca2+ has been implicated in several neurodegenerative diseases, while the catalogue of tumor suppressors includes a few that are enriched at the MAM.[38]
Molecular basis for tethering
Recent advances in the identification of the tethers between the mitochondrial and ER membranes suggest that the scaffolding function of the molecular elements involved is secondary to other, non-structural functions. In yeast, ERMES, a multiprotein complex of interacting ER- and mitochondrial-resident membrane proteins, is required for lipid transfer at the MAM and exemplifies this principle. One of its components, for example, is also a constituent of the protein complex required for insertion of transmembrane beta-barrel proteins into the lipid bilayer.[33] However, a homologue of the ERMES complex has not been identified yet in mammalian cells. Other proteins implicated in scaffolding likewise have functions independent of structural tethering at the MAM; for example, ER-resident and mitochondrial-resident mitofusins form heterocomplexes that regulate the number of inter-organelle contact sites, although mitofusins were first identified for their role in fission and fusion events between individual mitochondria.[27] Glucose-related protein 75 (grp75) is another dual-function protein. In addition to the matrix pool of grp75, a portion serves as a chaperone that physically links the mitochondrial and ER Ca2+ channels VDAC and IP3R for efficient Ca2+ transmission at the MAM.[27][28] Another potential tether is Sigma-1R, a non-opioid receptor whose stabilization of ER-resident IP3R may preserve communication at the MAM during the metabolic stress response.[40][41]

Perspective
The MAM is a critical signaling, metabolic, and trafficking hub in the cell that allows for the integration of ER and mitochondrial physiology. Coupling between these organelles is not simply structural but functional as well and critical for overall cellular physiology and homeostasis. The MAM thus offers a perspective on mitochondria that diverges from the traditional view of this organelle as a static, isolated unit appropriated for its metabolic capacity by the cell. Instead, this mitochondrial-ER interface emphasizes the integration of the mitochondria, the product of an endosymbiotic event, into diverse cellular processes.
Organization and distribution
Mitochondria are found in nearly all eukaryotes.[42] They vary in number and location according to cell type. A single mitochondrion is often found in unicellular organisms. Conversely, numerous mitochondria are found in human liver cells, with about 1000–2000 mitochondria per cell, making up 1/5 of the cell volume.[8] The mitochondrial content of otherwise similar cells can vary substantially in size and membrane potential,[43] with differences arising from sources including uneven partitioning at cell divisions, leading to extrinsic differences in ATP levels and downstream cellular processes.[44] The mitochondria can be found nestled between myofibrils of muscle or wrapped around the sperm flagellum.[8] Often they form a complex 3D branching network inside the cell with the cytoskeleton. The association with the cytoskeleton determines mitochondrial shape, which can affect the function as well.[45] Mitochondria in cells are always distributed along microtubules and the distribution of these organelles is also correlated with the endoplasmic reticulum.[46] Recent evidence suggests that vimentin, one of the components of the cytoskeleton, is also critical to the association with the cytoskeleton.[47]
Function
The most prominent roles of mitochondria are to produce the energy currency of the cell, ATP (i.e., phosphorylation of ADP), through respiration, and to regulate cellular metabolism.[9] The central set of reactions involved in ATP production are collectively known as the citric acid cycle, or the Krebs Cycle. However, the mitochondrion has many other functions in addition to the production of ATP.
Energy conversion
A dominant role for the mitochondria is the production of ATP, as reflected by the large number of proteins in the inner membrane for this task. This is done by oxidizing the major products of glucose, pyruvate, and NADH, which are produced in the cytosol.[9] This process of cellular respiration, also known as aerobic respiration, is dependent on the presence of oxygen. When oxygen is limited, the glycolytic products will be metabolized by anaerobic fermentation, a process that is independent of the mitochondria.[9] The production of ATP from glucose has an approximately 13-times higher yield during aerobic respiration compared to fermentation.[48] Recently it has been shown that plant mitochondria can produce a limited amount of ATP without oxygen by using the alternate substrate nitrite.[49]
Pyruvate and the citric acid cycle
Each pyruvate molecule produced by glycolysis is actively transported across the inner mitochondrial membrane, and into the matrix where it is oxidized and combined with coenzyme A to form CO2, acetyl-CoA, and NADH.[9]
The acetyl-CoA is the primary substrate to enter the citric acid cycle, also known as the tricarboxylic acid (TCA) cycle or Krebs cycle. The enzymes of the citric acid cycle are located in the mitochondrial matrix, with the exception of succinate dehydrogenase, which is bound to the inner mitochondrial membrane as part of Complex II.[50] The citric acid cycle oxidizes the acetyl-CoA to carbon dioxide, and, in the process, produces reduced cofactors (three molecules of NADH and one molecule of FADH2) that are a source of electrons for the electron transport chain, and a molecule of GTP (that is readily converted to an ATP).[9]
NADH and FADH2: the electron transport chain

The redox energy from NADH and FADH2 is transferred to oxygen (O2) in several steps via the electron transport chain. These energy-rich molecules are produced within the matrix via the citric acid cycle but are also produced in the cytoplasm by glycolysis. Reducing equivalents from the cytoplasm can be imported via the malate-aspartate shuttle system of antiporter proteins or feed into the electron transport chain using a glycerol phosphate shuttle.[9] Protein complexes in the inner membrane (NADH dehydrogenase (ubiquinone), cytochrome c reductase, and cytochrome c oxidase) perform the transfer and the incremental release of energy is used to pump protons (H+) into the intermembrane space. This process is efficient, but a small percentage of electrons may prematurely reduce oxygen, forming reactive oxygen species such as superoxide.[9] This can cause oxidative stress in the mitochondria and may contribute to the decline in mitochondrial function associated with the aging process.[51]
As the proton concentration increases in the intermembrane space, a strong electrochemical gradient is established across the inner membrane. The protons can return to the matrix through the ATP synthase complex, and their potential energy is used to synthesize ATP from ADP and inorganic phosphate (Pi).[9] This process is called chemiosmosis, and was first described by Peter Mitchell[52][53] who was awarded the 1978 Nobel Prize in Chemistry for his work. Later, part of the 1997 Nobel Prize in Chemistry was awarded to Paul D. Boyer and John E. Walker for their clarification of the working mechanism of ATP synthase.[54]
Heat production
Under certain conditions, protons can re-enter the mitochondrial matrix without contributing to ATP synthesis. This process is known as proton leak or mitochondrial uncoupling and is due to the facilitated diffusion of protons into the matrix. The process results in the unharnessed potential energy of the proton electrochemical gradient being released as heat.[9] The process is mediated by a proton channel called thermogenin, or UCP1.[55] Thermogenin is a 33kDa protein first discovered in 1973.[56] Thermogenin is primarily found in brown adipose tissue, or brown fat, and is responsible for non-shivering thermogenesis. Brown adipose tissue is found in mammals, and is at its highest levels in early life and in hibernating animals. In humans, brown adipose tissue is present at birth and decreases with age.[55]
Storage of calcium ions

The concentrations of free calcium in the cell can regulate an array of reactions and is important for signal transduction in the cell. Mitochondria can transiently store calcium, a contributing process for the cell's homeostasis of calcium.[57] In fact, their ability to rapidly take in calcium for later release makes them very good "cytosolic buffers" for calcium.[58][59][60] The endoplasmic reticulum (ER) is the most significant storage site of calcium, and there is a significant interplay between the mitochondrion and ER with regard to calcium.[61] The calcium is taken up into the matrix by a calcium uniporter on the inner mitochondrial membrane.[62] It is primarily driven by the mitochondrial membrane potential.[57] Release of this calcium back into the cell's interior can occur via a sodium-calcium exchange protein or via "calcium-induced-calcium-release" pathways.[62] This can initiate calcium spikes or calcium waves with large changes in the membrane potential. These can activate a series of second messenger system proteins that can coordinate processes such as neurotransmitter release in nerve cells and release of hormones in endocrine cells.
Ca2+ influx to the mitochondrial matrix has recently been implicated as a mechanism to regulate respiratory bioenergetics by allowing the electrochemical potential across the membrane to transiently "pulse" from ΔΨ-dominated to pH-dominated, facilitating a reduction of oxidative stress.[63] In neurons, concominant increases in cytosolic and mitochondrial calcium act to synchronize neuronal activity with mitochondrial energy metabolism. Mitochondrial matrix calcium levels can reach the tens of micromolar levels, which is necessary for the activation of isocitrate dehydrogenase, one of the key regulatory enzymes of the Kreb's cycle.[64]
Additional functions
Mitochondria play a central role in many other metabolic tasks, such as:
- Signaling through mitochondrial reactive oxygen species[65]
- Regulation of the membrane potential[9]
- Apoptosis-programmed cell death[66]
- Calcium signaling (including calcium-evoked apoptosis)[67]
- Regulation of cellular metabolism[68]
- Certain heme synthesis reactions[69] (see also: porphyrin)
- Steroid synthesis.[58]
- Hormonal signaling [70] Mitochondria are sensitive and responsive to hormones, in part by the action of mitochondrial estrogen receptors (mtERs). These receptors have been found in various tissues and cell types, including brain [71] and heart [72]
Some mitochondrial functions are performed only in specific types of cells. For example, mitochondria in liver cells contain enzymes that allow them to detoxify ammonia, a waste product of protein metabolism. A mutation in the genes regulating any of these functions can result in mitochondrial diseases.
Cellular proliferation regulation
The relationship between cellular proliferation and mitochondria has been investigated using cervical cancer Hela cells. Tumor cells require an ample amount of ATP (Adenosine triphosphate) in order to synthesize bioactive compounds such as lipids, proteins, and nucleotides for rapid cell proliferation.[73] The majority of ATP in tumor cells is generated via the Oxidative Phosphorylation pathway (OxPhos).[74] Interference with OxPhos have shown to cause cell cycle arrest suggesting that mitochondria play a role in cell proliferation.[74] Mitochondrial ATP production is also vital for cell division in addition to other basic functions in the cell including the regulation of cell volume, solute concentration, and cellular architecture.[75][76][77] ATP levels differ at various stages of the cell cycle suggesting that there is a relationship between the abundance of ATP and the cell's ability to enter a new cell cycle.[78] ATP's role in the basic functions of the cell make the cell cycle sensitive to changes in the availability of mitochondrial derived ATP.[78] The variation in ATP levels at different stages of the cell cycle support the hypothesis that mitochondria play an important role in cell cycle regulation.[78] Although the specific mechanisms between mitochondria and the cell cycle regulation is not well understood, studies have shown that low energy cell cycle checkpoints monitor the energy capability before committing to another round of cell division.[79]
Origin
There are two hypotheses about the origin of mitochondria: endosymbiotic and autogenous. The endosymbiotic hypothesis suggests mitochondria were originally prokaryotic cells, capable of implementing oxidative mechanisms that were not possible to eukaryotic cells; they became endosymbionts living inside the eukaryote. In the autogenous hypothesis, mitochondria were born by splitting off a portion of DNA from the nucleus of the eukaryotic cell at the time of divergence with the prokaryotes; this DNA portion would have been enclosed by membranes, which could not be crossed by proteins. Since mitochondria have many features in common with bacteria, the most accredited theory at present is endosymbiosis.[80]
A mitochondrion contains DNA, which is organized as several copies of a single, circular chromosome. This mitochondrial chromosome contains genes for redox proteins such as those of the respiratory chain. The CoRR hypothesis proposes that this co-location is required for redox regulation. The mitochondrial genome codes for some RNAs of ribosomes, and the twenty-two tRNAs necessary for the translation of messenger RNAs into protein. The circular structure is also found in prokaryotes. The proto-mitochondrion was probably closely related to the Rickettsia.[81] However, the exact relationship of the ancestor of mitochondria to the alphaproteobacteria and whether the mitochondrion was formed at the same time or after the nucleus, remains controversial.[82]
A recent study[83] by researchers of the University of Hawaii at Manoa and the Oregon State University indicates that the SAR11 clade of bacteria shares a relatively recent common ancestor with the mitochondria existing in most eukaryotic cells.
Schematic ribosomal RNA phylogeny of Alphaproteobacteria | ||||||||||||||||||||||||
| ||||||||||||||||||||||||
The cladogram of Rickettsidae has been inferred by Ferla et al. [84] from the comparison of 16S + 23S ribosomal RNA sequences. |
The ribosomes coded for by the mitochondrial DNA are similar to those from bacteria in size and structure.[85] They closely resemble the bacterial 70S ribosome and not the 80S cytoplasmic ribosomes, which are coded for by nuclear DNA.
The endosymbiotic relationship of mitochondria with their host cells was popularized by Lynn Margulis.[86] The endosymbiotic hypothesis suggests that mitochondria descended from bacteria that somehow survived endocytosis by another cell, and became incorporated into the cytoplasm. The ability of these bacteria to conduct respiration in host cells that had relied on glycolysis and fermentation would have provided a considerable evolutionary advantage. This symbiotic relationship probably developed 1.7[87] to 2[88] billion years ago.
A few groups of unicellular eukaryotes lack mitochondria: the microsporidians, metamonads, and archamoebae.[89] These groups appear as the most primitive eukaryotes on phylogenetic trees constructed using rRNA information, which once suggested that they appeared before the origin of mitochondria. However, this is now known to be an artifact of long-branch attraction—they are derived groups and retain genes or organelles derived from mitochondria (e.g., mitosomes and hydrogenosomes).[1]
Genome

The human mitochondrial genome is a circular DNA molecule of about 16 kilobases.[90] It encodes 37 genes: 13 for subunits of respiratory complexes I, III, IV and V, 22 for mitochondrial tRNA (for the 20 standard amino acids, plus an extra gene for leucine and serine), and 2 for rRNA.[90] One mitochondrion can contain two to ten copies of its DNA.[91]
As in prokaryotes, there is a very high proportion of coding DNA and an absence of repeats. Mitochondrial genes are transcribed as multigenic transcripts, which are cleaved and polyadenylated to yield mature mRNAs. Not all proteins necessary for mitochondrial function are encoded by the mitochondrial genome; most are coded by genes in the cell nucleus and the corresponding proteins are imported into the mitochondrion.[26] The exact number of genes encoded by the nucleus and the mitochondrial genome differs between species. Most mitochondrial genomes are circular, although exceptions have been reported.[92] In general, mitochondrial DNA lacks introns, as is the case in the human mitochondrial genome;[26] however, introns have been observed in some eukaryotic mitochondrial DNA,[93] such as that of yeast[94] and protists,[95] including Dictyostelium discoideum.[96] Between protein-coding regions, tRNAs are present. During transcription, the tRNAs acquire their characteristic L-shape that gets recognized and cleave by specific enzymes. Mitochondrial tRNA genes have different sequences from the nuclear tRNAs but lookalikes of mitochondrial tRNAs have been found in the nuclear chromosomes with high sequence similarity. [97]
In animals the mitochondrial genome is typically a single circular chromosome that is approximately 16 kb long and has 37 genes. The genes, while highly conserved, may vary in location. Curiously, this pattern is not found in the human body louse (Pediculus humanus). Instead this mitochondrial genome is arranged in 18 minicircular chromosomes, each of which is 3–4 kb long and has one to three genes.[98] This pattern is also found in other sucking lice, but not in chewing lice. Recombination has been shown to occur between the minichromosomes. The reason for this difference is not known.
While slight variations on the standard code had been predicted earlier,[99] none was discovered until 1979, when researchers studying human mitochondrial genes determined that they used an alternative code.[100] Although, the mitochondria of many other eukaryotes, including most plants, use the standard code.[101] Many slight variants have been discovered since,[102] including various alternative mitochondrial codes.[103] Further, the AUA, AUC, and AUU codons are all allowable start codons.
Organism | Codon | Standard | Mitochondria |
---|---|---|---|
Mammals | AGA, AGG | Arginine | Stop codon |
Invertebrates | AGA, AGG | Arginine | Serine |
Fungi | CUA | Leucine | Threonine |
All of the above | AUA | Isoleucine | Methionine |
UGA | Stop codon | Tryptophan |
Some of these differences should be regarded as pseudo-changes in the genetic code due to the phenomenon of RNA editing, which is common in mitochondria. In higher plants, it was thought that CGG encoded for tryptophan and not arginine; however, the codon in the processed RNA was discovered to be the UGG codon, consistent with the standard genetic code for tryptophan.[104] Of note, the arthropod mitochondrial genetic code has undergone parallel evolution within a phylum, with some organisms uniquely translating AGG to lysine.[105]
Mitochondrial genomes have far fewer genes than the bacteria from which they are thought to be descended. Although some have been lost altogether, many have been transferred to the nucleus, such as the respiratory complex II protein subunits.[90] This is thought to be relatively common over evolutionary time. A few organisms, such as the Cryptosporidium, actually have mitochondria that lack any DNA, presumably because all their genes have been lost or transferred.[106] In Cryptosporidium, the mitochondria have an altered ATP generation system that renders the parasite resistant to many classical mitochondrial inhibitors such as cyanide, azide, and atovaquone.[106]
Replication and inheritance
Mitochondria divide by binary fission, similar to bacterial cell division.[107] The regulation of this division differs between eukaryotes. In many single-celled eukaryotes, their growth and division is linked to the cell cycle. For example, a single mitochondrion may divide synchronously with the nucleus. This division and segregation process must be tightly controlled so that each daughter cell receives at least one mitochondrion. In other eukaryotes (in mammals for example), mitochondria may replicate their DNA and divide mainly in response to the energy needs of the cell, rather than in phase with the cell cycle. When the energy needs of a cell are high, mitochondria grow and divide. When the energy use is low, mitochondria are destroyed or become inactive. In such examples, and in contrast to the situation in many single celled eukaryotes, mitochondria are apparently randomly distributed to the daughter cells during the division of the cytoplasm. Understanding of mitochondrial dynamics, which is described as the balance between mitochondrial fusion and fission, has revealed that functional and structural alterations in mitochondrial morphology are important factors in pathologies associated with several disease conditions.[108]
It is worthwhile to point out that the hypothesis of mitochondrial binary fission has relied on the visualization by fluorescence microscopy and conventional transmission electron microscopy (TEM). As is well known, the resolution of fluorescence microscopy(~200 nm) is insufficient to distinguish structural details such as double mitochondrial membrane in mitochondrial division. It is even not sufficient to distinguish individual mitochondrion when multiple mitochondria are close to each other. Conventional TEM has also some technical limitations in verifying mitochondrial division. A cutting edge technique termed Cryo-electron tomography was recently utilized to visualize mitochondrial division in frozen hydrated intact cells. It revealed that mitochondria divide by budding.[109]
An individual's mitochondrial genes are not inherited by the same mechanism as nuclear genes. Typically, the mitochondria are inherited from one parent only. In humans, when an egg cell is fertilized by a sperm, the egg nucleus and sperm nucleus each contribute equally to the genetic makeup of the zygote nucleus. In contrast, the mitochondria, and therefore the mitochondrial DNA, usually come from the egg only. The sperm's mitochondria enter the egg but do not contribute genetic information to the embryo.[110] Instead, paternal mitochondria are marked with ubiquitin to select them for later destruction inside the embryo.[111] The egg cell contains relatively few mitochondria, but it is these mitochondria that survive and divide to populate the cells of the adult organism. Mitochondria are, therefore, in most cases inherited only from mothers, a pattern known as maternal inheritance. This mode is seen in most organisms including the majority of animals. However, mitochondria in some species can sometimes be inherited paternally. This is the norm among certain coniferous plants, although not in pine trees and yew trees.[112] For Mytilidae mussels paternal inheritance only occurs within males of the species.[113][114][115] It has been suggested that it occurs at a very low level in humans.[116] There is a recent suggestion mitochondria that shorten male lifespan stay in the system because mitochondria are inherited only through the mother. By contrast natural selection weeds out mitochondria that reduce female survival as such mitochondria are less likely to be passed on to the next generation. Therefore it is suggested human females and female animals tend to live longer than males. The authors claim this is a partial explanation.[117]
Uniparental inheritance leads to little opportunity for genetic recombination between different lineages of mitochondria, although a single mitochondrion can contain 2–10 copies of its DNA.[91] For this reason, mitochondrial DNA usually is thought to reproduce by binary fission. What recombination does take place maintains genetic integrity rather than maintaining diversity. However, there are studies showing evidence of recombination in mitochondrial DNA. It is clear that the enzymes necessary for recombination are present in mammalian cells.[118] Further, evidence suggests that animal mitochondria can undergo recombination.[119] The data are a bit more controversial in humans, although indirect evidence of recombination exists.[120][121] If recombination does not occur, the whole mitochondrial DNA sequence represents a single haplotype, which makes it useful for studying the evolutionary history of populations.
Population genetic studies
The near-absence of genetic recombination in mitochondrial DNA makes it a useful source of information for scientists involved in population genetics and evolutionary biology.[122] Because all the mitochondrial DNA is inherited as a single unit, or haplotype, the relationships between mitochondrial DNA from different individuals can be represented as a gene tree. Patterns in these gene trees can be used to infer the evolutionary history of populations. The classic example of this is in human evolutionary genetics, where the molecular clock can be used to provide a recent date for mitochondrial Eve.[123][124] This is often interpreted as strong support for a recent modern human expansion out of Africa.[125] Another human example is the sequencing of mitochondrial DNA from Neanderthal bones. The relatively large evolutionary distance between the mitochondrial DNA sequences of Neanderthals and living humans has been interpreted as evidence for lack of interbreeding between Neanderthals and anatomically modern humans.[126]
However, mitochondrial DNA reflects the history of only females in a population and so may not represent the history of the population as a whole. This can be partially overcome by the use of paternal genetic sequences, such as the non-recombining region of the Y-chromosome.[125] In a broader sense, only studies that also include nuclear DNA can provide a comprehensive evolutionary history of a population.[127]
Dysfunction and disease
Mitochondrial diseases
Damage and subsequent dysfunction in mitochondria is an important factor in a range of human diseases due to their influence in cell metabolism. Mitochondrial disorders often present themselves as neurological disorders, including autism.[7] They can also manifest as myopathy, diabetes, multiple endocrinopathy, and a variety of other systemic disorders.[128] Diseases caused by mutation in the mtDNA include Kearns-Sayre syndrome, MELAS syndrome and Leber's hereditary optic neuropathy.[129] In the vast majority of cases, these diseases are transmitted by a female to her children, as the zygote derives its mitochondria and hence its mtDNA from the ovum. Diseases such as Kearns-Sayre syndrome, Pearson's syndrome, and progressive external ophthalmoplegia are thought to be due to large-scale mtDNA rearrangements, whereas other diseases such as MELAS syndrome, Leber's hereditary optic neuropathy, myoclonic epilepsy with ragged red fibers (MERRF), and others are due to point mutations in mtDNA.[128]
In other diseases, defects in nuclear genes lead to dysfunction of mitochondrial proteins. This is the case in Friedreich's ataxia, hereditary spastic paraplegia, and Wilson's disease.[130] These diseases are inherited in a dominance relationship, as applies to most other genetic diseases. A variety of disorders can be caused by nuclear mutations of oxidative phosphorylation enzymes, such as coenzyme Q10 deficiency and Barth syndrome.[128] Environmental influences may interact with hereditary predispositions and cause mitochondrial disease. For example, there may be a link between pesticide exposure and the later onset of Parkinson's disease.[131][132] Other pathologies with etiology involving mitochondrial dysfunction include schizophrenia, bipolar disorder, dementia, Alzheimer's disease,[133] Parkinson's disease, epilepsy, stroke, cardiovascular disease, retinitis pigmentosa, and diabetes mellitus.[134][135]
Mitochondria-mediated oxidative stress plays a role in cardiomyopathy in Type 2 diabetics. Increased fatty acid delivery to the heart increases fatty acid uptake by cardiomyocytes, resulting in increased fatty acid oxidation in these cells. This process increases the reducing equivalents available to the electron transport chain of the mitochondria, ultimately increasing reactive oxygen species (ROS) production. ROS increases uncoupling proteins (UCPs) and potentiate proton leakage through the adenine nucleotide translocator (ANT), the combination of which uncouples the mitochondria. Uncoupling then increases oxygen consumption by the mitochondria, compounding the increase in fatty acid oxiation. This creates a vicious cycle of uncoupling; furthermore, even though oxygen consumption increases, ATP synthesis does not increase proportionally because the mitochondria is uncoupled. Less ATP availability ultimately results in an energy deficit presenting as reduced cardiac efficiency and contractile dysfunction. To compound the problem, impaired sarcoplasmic reticulum calcium release and reduced mitochondrial reuptake limits peak cytosolic levels of the important signaling ion during muscle contraction. The decreased intra-mitochondrial calcium concentration increases dehydrogenase activation and ATP synthesis. So in addition to lower ATP synthesis due to fatty acid oxidation, ATP synthesis is impaired by poor calcium signaling as well, causing cardiac problems for diabetics.[136]
Possible relationships to aging
Given the role of mitochondria as the cell's powerhouse, there may be some leakage of the high-energy electrons in the respiratory chain to form reactive oxygen species. This was thought to result in significant oxidative stress in the mitochondria with high mutation rates of mitochondrial DNA (mtDNA).[137] Hypothesized links between aging and oxidative stress are not new and were proposed in 1956,[138] which was later refined into the mitochondrial free radical theory of aging.[139] A vicious cycle was thought to occur, as oxidative stress leads to mitochondrial DNA mutations, which can lead to enzymatic abnormalities and further oxidative stress. However, recent measurements of the rate of accumulation of mutation observed in mitochondrial DNA[140] were estimated to be 1 mutation every 7884 years (10−7 to 10−9 per base per year, dating back to the most recent common ancestor of humans and apes), consistent with other estimates of mutation rates of autosomal dna ( 10−8 per base per generation[141])
A number of changes can occur to mitochondria during the aging process.[142] Tissues from elderly patients show a decrease in enzymatic activity of the proteins of the respiratory chain.[143] However, mutated mtDNA can only be found in about 0.2% of very old cells.[144] Large deletions in the mitochondrial genome have been hypothesized to lead to high levels of oxidative stress and neuronal death in Parkinson's disease.[145] However, there is much debate over whether mitochondrial changes are causes of aging or merely characteristics of aging. One notable study in mice demonstrated shortened lifespan but no increase in reactive oxygen species despite increasing mitochondrial DNA mutations.[146] However, it has to be noted that aging non-mutant mice do not seem to accumulate a great number of mutations in mitochondrial DNA imposing a cloud of doubt on the involvement of mitochondrial DNA mutations in "natural" aging. As a result, the exact relationships between mitochondria, oxidative stress, and aging have not yet been settled.
See also
References
- ^ a b Henze K, Martin W; Martin, William (2003). "Evolutionary biology: essence of mitochondria". Nature. 426 (6963): 127–8. doi:10.1038/426127a. PMID 14614484.
- ^ "mitochondria". Online Etymology Dictionary.
- ^ Campbell, Neil A.; Brad Williamson; Robin J. Heyden (2006). Biology: Exploring Life. Boston, Massachusetts: Pearson Prentice Hall. ISBN 0-13-250882-6.
- ^ McBride HM, Neuspiel M, Wasiak S (2006). "Mitochondria: more than just a powerhouse". Curr. Biol. 16 (14): R551–60. doi:10.1016/j.cub.2006.06.054. PMID 16860735.
{{cite journal}}
: CS1 maint: multiple names: authors list (link) - ^ Gardner A, Boles RG (2005). "Is a "Mitochondrial Psychiatry" in the Future? A Review". Curr. Psychiatry Review. 1 (3): 255–271. doi:10.2174/157340005774575064.
- ^ Lesnefsky EJ, Moghaddas S, Tandler B, Kerner B, Hoppel CL (June 2001). "Mitochondrial dysfunction in cardiac disease: ischemia—reperfusion, aging, and heart failure". Journal of Molecular and Cellular Cardiology. 33 (6): 1065–1089. doi:10.1006/jmcc.2001.1378. PMID 11444914.
{{cite journal}}
: CS1 maint: multiple names: authors list (link) - ^ a b http://www.biosciencetechnology.com/news/2014/05/study-confirms-mitochondrial-deficits-children-autism
- ^ a b c d e f g h i j k l Alberts, Bruce; Alexander Johnson; Julian Lewis; Martin Raff; Keith Roberts; Peter Walter (1994). Molecular Biology of the Cell. New York: Garland Publishing Inc. ISBN 0-8153-3218-1.
- ^ a b c d e f g h i j k Voet, Donald; Judith G. Voet; Charlotte W. Pratt (2006). Fundamentals of Biochemistry, 2nd Edition. John Wiley and Sons, Inc. p. 547. ISBN 0-471-21495-7.
- ^ Taylor SW, Fahy E, Zhang B, Glenn GM, Warnock DE, Wiley S, Murphy AN, Gaucher SP, Capaldi RA, Gibson BW, Ghosh SS (March 2003). "Characterization of the human heart mitochondrial proteome". Nat Biotechnol. 21 (3): 281–6. doi:10.1038/nbt793. PMID 12592411.
{{cite journal}}
: CS1 maint: multiple names: authors list (link) - ^ Zhang J, Li X, Mueller M, Wang Y, Zong C, Deng N, Vondriska TM, Liem DA, Yang J, Korge P, Honda H, Weiss JN, Apweiler R, Ping P (2008). "Systematic Characterization of the Murine Mitochondrial Proteome Using Functionally Validated Cardiac Mitochondria". Proteomics. 8 (8): 1564–1575. doi:10.1002/pmic.200700851. PMC 2799225. PMID 18348319.
{{cite journal}}
: CS1 maint: multiple names: authors list (link) - ^ Zhang J, Liem DA, Mueller M, Wang Y, Zong C, Deng N, Vondriska TM, Yang J, Korge P, Drews O, Maclellan WR, Honda H, Weiss JN, Apweiler R, Ping P (2008). "Altered Proteome Biology of Cardiac Mitochondria Under Stress Conditions". J. Proteome Res. 7 (6): 2204–14. doi:10.1021/pr070371f. PMID 18484766.
{{cite journal}}
: CS1 maint: multiple names: authors list (link) - ^ Andersson SG, Karlberg O, Canbäck B, Kurland CG (January 2003). "On the origin of mitochondria: a genomics perspective". Philosophical Transactions of the Royal Society B. 358 (1429): 165–77, discussion 177–9. doi:10.1098/rstb.2002.1193. PMC 1693097. PMID 12594925.
{{cite journal}}
: CS1 maint: multiple names: authors list (link) - ^ a b c d e f g Ernster L, Schatz G (1981). "Mitochondria: a historical review". The Journal of Cell Biology. 91 (3 Pt 2): 227s – 255s. doi:10.1083/jcb.91.3.227s. PMC 2112799. PMID 7033239.
- ^ Richard Altmann, Die Elementarorganismen und ihre Beziehungen zu den Zellen [The elementary organisms and their relations to cells], (Leipzig, Germany: Veit & Co., 1890), p. 125. From p. 125: "Da auch sonst mancherlei Umstände dafür sprechen, dass Mikroorganismen und Granula einander gleichwerthig sind und Elementarorganismen vorstellen, welche sich überall finden, wo lebendige Kräfte ausgelöst werden, so wollen wir sie mit dem gemeinschaftlichen Namen der Bioblasten bezeichnen." (Since also in other ways various circumstances indicate that microörganisms and granula are equivalent to each other and represent elementary organisms, which are found wherever living forces are initiated, then we will designate them with the common name of "bioblasts". [Altmann believed that mitochondria were bacteria that lived symbiotically in the cells of all eukaryotic organisms.]) Available on-line at: Deutsches Textarchiv, Berlin
- ^ Benda, C. (1898) "Ueber die Spermatogenese der Vertebraten und höherer Evertebraten, II. Theil: Die Histiogenese der Spermien" (On spermatogenesis of vertebrates and higher invertebrates, Part II: The histogenesis of sperm), Archiv für Anatomie und Physiologie, 73 : 393-398 ; see especially p. 397. From p. 397: "Sie liegen innerhalb eines Theiles der Protoplasmafäden, bisweilen zu besonderen Körpern gehäuft, und sind wenigstens mit einem Theil der bereits bekannten Zellmikrosomen identisch, aber unterschieden von den Altmann'schen und Ehrlich'schen Granulationen. Ich möchte vorläufig vorschlagen, ihnen als Mitochondria eine besondere Stellung vorzubehalten, die ich in weiteren Arbeiten begründen werde." (They lie within of a portion of the protoplasmic threads, sometimes aggregated to particular bodies, and are identical to at least a portion of the already known cell microsomes, but differ from Altmann's and Ehrlich's granulations. I would like to suggest tentatively reserving for them a special status as "mitochondria", which I will justify in further work.)
- ^ Fr. Meves (June 1904) "Über das Vorkommen von Mitochondrien bezw. Chondromiten in Pflanzenzellen" (On the occurrence of mitochondria or chondromites in plant cells), Berichte der Deutschen Botanischen Gesellschaft (Reports of the German Botanical Society), 22 (5) : 284–286.
- ^ Siekevitz P (1957). "Powerhouse of the cell". Scientific American. 197: 131–140. doi:10.1038/scientificamerican0757-131.
- ^ "Mitochondrion – much more than an energy converter". British Society for Cell Biology. Retrieved 19 August 2013.
- ^ a b Herrmann JM, Neupert W (April 2000). "Protein transport into mitochondria". Current Opinion in Microbiology. 3 (2): 210–214. doi:10.1016/S1369-5274(00)00077-1. PMID 10744987.
- ^ a b Chipuk JE, Bouchier-Hayes L, Green DR (2006). "Mitochondrial outer membrane permeabilization during apoptosis: the innocent bystander scenario". Cell Death and Differentiation. 13 (8): 1396–1402. doi:10.1038/sj.cdd.4401963. PMID 16710362.
{{cite journal}}
: CS1 maint: multiple names: authors list (link) - ^ Hayashi T, Rizzuto R, Hajnoczky G, Su TP (February 2009). "MAM: more than just a housekeeper". Trends Cell Biol. 19 (2): 81–8. doi:10.1016/j.tcb.2008.12.002. PMC 2750097. PMID 19144519.
{{cite journal}}
: CS1 maint: multiple names: authors list (link) - ^ McMillin JB, Dowhan W (December 2002). "Cardiolipin and apoptosis". Biochim. Et Biophys. Acta. 1585 (2–3): 97–107. doi:10.1016/S1388-1981(02)00329-3. PMID 12531542.
- ^ Mannella CA (2006). "Structure and dynamics of the mitochondrial inner membrane cristae". Biochimica et Biophysica Acta. 1763 (5–6): 542–548. doi:10.1016/j.bbamcr.2006.04.006. PMID 16730811.
- ^ Thar, R.; Kühl, Michael (2004). "Propagation of electromagetic radiation in mitochondria?" (PDF). J Theor Biol. 230 (2): 261–270. doi:10.1016/j.jtbi.2004.05.021.
- ^ a b c Anderson S, Bankier AT, Barrell BG, de-Bruijn MHL, Coulson AR; et al. (1981). "Sequence and organization of the human mitochondrial genome". Nature. 290 (5806): 427–465. doi:10.1038/290457a0. PMID 7219534.
{{cite journal}}
: Explicit use of et al. in:|author=
(help)CS1 maint: multiple names: authors list (link) - ^ a b c d e f g h i j k l m n Rizzuto, R.; Marchi, Saverio; Bonora, Massimo; Aguiari, Paola; Bononi, Angela; De Stefani, Diego; Giorgi, Carlotta; Leo, Sara; Rimessi, Alessandro; et al. (2009). "Ca2+ transfer from the ER to mitochondria: when, how and why". Biochim Biophys Acta. 1787 (11): 1342–51. doi:10.1016/j.bbabio.2009.03.015. PMC 2730423. PMID 19341702.
{{cite journal}}
: Explicit use of et al. in:|author=
(help); Unknown parameter|displayauthors=
ignored (|display-authors=
suggested) (help) - ^ a b c d Hayashi, T.; Rizzuto, Rosario; Hajnoczky, Gyorgy; Su, Tsung-Ping; et al. (2009). "MAM: more than just a housekeeper". Trends Cell Biol. 19 (2): 81–88. doi:10.1016/j.tcb.2008.12.002. PMC 2750097. PMID 19144519.
{{cite journal}}
: Explicit use of et al. in:|author=
(help) - ^ a b de Brito, OM; et al. (2010). "An intimate liaison: spatial organization of the endoplasmic reticulum–mitochondria relationship". EMBO J. 29 (16): 2715–2723. doi:10.1038/emboj.2010.177. PMC 2924651. PMID 20717141.
{{cite journal}}
: Explicit use of et al. in:|author=
(help) - ^ a b Vance, JE.; Shiao, YJ; et al. (1996). "Intracellular trafficking of phospholipids: import of phosphatidylserine into mitochondria". Anticancer Research. 16 (3B): 1333–9. PMID 8694499.
{{cite journal}}
: Explicit use of et al. in:|author=
(help) - ^ a b c Lebiedzinska, M; Szabadkai, György; Jones, Aleck W.E.; Duszynski, Jerzy; Wieckowski, Mariusz R.; et al. (2009). "Interactions between the endoplasmic reticulum, mitochondria, plasma membrane and other subcellular organelles". Int J Biochem Cell Biol. 41 (10): 1805–16. doi:10.1016/j.biocel.2009.02.017. PMID 19703651.
{{cite journal}}
: Explicit use of et al. in:|author=
(help) - ^ Twig, G; Elorza, Alvaro; Molina, Anthony J A; Mohamed, Hibo; Wikstrom, Jakob D; Walzer, Gil; Stiles, Linsey; Haigh, Sarah E; Katz, Steve; et al. (2008). "Fission and selective fusion govern mitochondrial segregation and elimination by autophagy". The EMBO Journal. 27 (2): 433–446. doi:10.1038/sj.emboj.7601963. PMC 2234339. PMID 18200046.
{{cite journal}}
: Explicit use of et al. in:|author=
(help); Unknown parameter|displayauthors=
ignored (|display-authors=
suggested) (help) - ^ a b c d e f Osman, C; Voelker, D. R.; Langer, T.; et al. (2011). "Making heads or tails of phospholipids in mitochondria". J Cell Biol. 192 (1): 7–16. doi:10.1083/jcb.201006159. PMC 3019561. PMID 21220505.
{{cite journal}}
: Explicit use of et al. in:|author=
(help) - ^ Kornmann, B.; Currie, E.; Collins, S. R.; Schuldiner, M.; Nunnari, J.; Weissman, J. S.; Walter, P.; et al. (2009). "An ER-Mitochondria Tethering Complex Revealed by a Synthetic Biology Screen". Science. 325 (24): 477–481. doi:10.1126/science.1175088. PMC 2933203. PMID 19556461.
{{cite journal}}
: Explicit use of et al. in:|author=
(help) - ^ Rusinol, AE; Cui, Z; Chen, MH; Vance, JE; et al. (1994). "A Unique Mitochondria-associated Membrane Fraction from Rat Liver Has a High Capacity for Lipid Synthesis and Contains Pre-Golgi Secretory Proteins Including Nascent Lipoprotein". J Biol Chem. 269 (44): 27494–27502. PMID 7961664.
{{cite journal}}
: Explicit use of et al. in:|author=
(help) - ^ a b Kopach, O; Kruglikov, Illya; Pivneva, Tatyana; Voitenko, Nana; Fedirko, Nataliya; et al. (2008). "Functional coupling between ryanodine receptors, mitochondria and Ca2+ ATPases in rat submandibular acinar cells". Cell Calcium. 43 (5): 469–481. doi:10.1016/j.ceca.2007.08.001. PMID 17889347.
{{cite journal}}
: Explicit use of et al. in:|author=
(help) - ^ Csordas, G; Hajnóczky, G; et al. (2001). "Sorting of calcium signals at the junctions of endoplasmic reticulum and mitochondria". Cell Calcium. 29 (4): 249–262. doi:10.1054/ceca.2000.0191. PMID 11243933.
{{cite journal}}
: Explicit use of et al. in:|author=
(help) - ^ a b c d Decuypere, JP; Monaco, Giovanni; Bultynck, Geert; Missiaen, Ludwig; De Smedt, Humbert; Parys, Jan B.; et al. (2011). "The IP3 receptor–mitochondria connection in apoptosis and autophagy". Biochim Biophys Acta. 1813 (5): 1003–13. doi:10.1016/j.bbamcr.2010.11.023. PMID 21146562.
{{cite journal}}
: Explicit use of et al. in:|author=
(help) - ^ Hajnoczky, G.; Csordás, G; Yi, M; et al. (2011). "Old players in a new role: mitochondria-associated membranes, VDAC, and ryanodine receptors as contributors to calcium signal propagation from endoplasmic reticulum to the mitochondria". Cell Calcium. 32 (5–6): 363–377. doi:10.1016/S0143416002001872. PMID 12543096.
{{cite journal}}
: Explicit use of et al. in:|author=
(help) - ^ Marriott, KS; Prasad, M; Thapliyal, V; Bose, HS (December 2012). "σ-1 Receptor at the Mitochondrial-Associated Endoplasmic Reticulum Membrane Is Responsible for Mitochondrial Metabolic Regulation". The Journal of Pharmacology and Experimental Therapeutics. 343 (3): 578–86. doi:10.1124/jpet.112.198168. PMC 3500540. PMID 22923735.
- ^ Hayashi, T; Su, TP (Nov 2, 2007). "Sigma-1 receptor chaperones at the ER-mitochondrion interface regulate Ca(2+) signaling and cell survival". Cell. 131 (3): 596–610. doi:10.1016/j.cell.2007.08.036. PMID 17981125.
- ^ The eukaryote Giardia lamblia, for example, does not contain mitochondria, but does have a mitochondiral-like gene, suggesting that it once included either mitochondria or an endosymbiotic progenitor of it Roger, Andrew J.; Svärd, Staffan G.; Tovar, Jorge; Clark, C. Graham; Smith, Michael W. Smith, Gillin, Frances D., and Sogin, Mitchell L. (1998). "A mitochondrial-like chaperonin 60 gene in Giardia lamblia: Evidence that diplomonads once harbored an endosymbiont related to the progenitor of mitochondria". Proceedings of the National Academy of Sciences. 95 (1). National Academy of Sciences: 229–234. doi:10.1073/pnas.95.1.229. Retrieved June 1, 2013.
{{cite journal}}
: CS1 maint: multiple names: authors list (link) - ^ das Neves RP, Jones NS, Andreu L, Gupta R, Enver T, Iborra FJ (2010). Weissman, Jonathan S (ed.). "Connecting Variability in Global Transcription Rate to Mitochondrial Variability". PLOS Biology. 8 (12): e1000560. doi:10.1371/journal.pbio.1000560. PMC 3001896. PMID 21179497.
{{cite journal}}
: CS1 maint: multiple names: authors list (link) CS1 maint: unflagged free DOI (link) - ^ Johnston IG, Gaal B, das Neves RP, Enver T, Iborra FJ, Jones NS (2012). Haugh, Jason M (ed.). "Mitochondrial Variability as a Source of Extrinsic Cellular Noise". PLOS Computational Biology. 8 (3): e1002416. doi:10.1371/journal.pcbi.1002416. PMC 3297557. PMID 22412363.
{{cite journal}}
: CS1 maint: multiple names: authors list (link) CS1 maint: unflagged free DOI (link) - ^ Rappaport L, Oliviero P, Samuel JL (1998). "Cytoskeleton and mitochondrial morphology and function". Mol and Cell Biochem. 184: 101–105. doi:10.1023/A:1006843113166.
{{cite journal}}
: CS1 maint: multiple names: authors list (link) - ^ Soltys, B.J. and Gupta, R.S (1992) Interrelationships of endoplasmic reticulum, mitochondria, intermediate filaments and microtubules - a quadruple fluorescence labeling study. Biochem. Cell Biol. 70: 1174-1186.
- ^ Tang HL, Lung HL, Wu KC, Le AP, Tang HM, Fung MC (2007). "Vimentin supports mitochondrial morphology and organization". Biochemical J. 410 (1): 141–6. doi:10.1042/BJ20071072. PMID 17983357.
{{cite journal}}
: CS1 maint: multiple names: authors list (link) - ^ Rich PR (2003). "The molecular machinery of Keilin's respiratory chain". Biochem. Soc. Trans. 31 (Pt 6): 1095–105. doi:10.1042/BST0311095. PMID 14641005.
- ^ Stoimenova M, Igamberdiev AU, Gupta KJ, Hill RD (July 2007). "Nitrite-driven anaerobic ATP synthesis in barley and rice root mitochondria". Planta. 226 (2): 465–74. doi:10.1007/s00425-007-0496-0. PMID 17333252.
{{cite journal}}
: CS1 maint: multiple names: authors list (link) - ^ King A, Selak MA, Gottlieb E (2006). "Succinate dehydrogenase and fumarate hydratase: linking mitochondrial dysfunction and cancer". Oncogene. 25 (34): 4675–4682. doi:10.1038/sj.onc.1209594. PMID 16892081.
{{cite journal}}
: CS1 maint: multiple names: authors list (link) - ^ Huang, K.; K. G. Manton (2004). "The role of oxidative damage in mitochondria during aging: A review". Frontiers in Bioscience. 9: 1100–1117. doi:10.2741/1298. PMID 14977532.
- ^ Mitchell P, Moyle J (1967-01-14). "Chemiosmotic hypothesis of oxidative phosphorylation". Nature. 213 (5072): 137–9. doi:10.1038/213137a0. PMID 4291593.
- ^ Mitchell P (1967-06-24). "Proton current flow in mitochondrial systems". Nature. 25 (5095): 1327–8. doi:10.1038/2141327a0. PMID 6056845.
- ^ Nobel Foundation. "Chemistry 1997". Retrieved 2007-12-16.
- ^ a b Mozo J, Emre Y, Bouillaud F, Ricquier D, Criscuolo F (November 2005). "Thermoregulation: What Role for UCPs in Mammals and Birds?". Bioscience Reports. 25 (3–4): 227–249. doi:10.1007/s10540-005-2887-4. PMID 16283555.
{{cite journal}}
: CS1 maint: multiple names: authors list (link) - ^ Nicholls DG, Lindberg O (1973). "Brown-adipose-tissue mitochondria. The influence of albumin and nucleotides on passive ion permeabilities". Eur. J. Biochem. 37 (3): 523–30. doi:10.1111/j.1432-1033.1973.tb03014.x. PMID 4777251.
- ^ a b Editor-in-chief, George J. Siegel; editors, Bernard W. Agranoff... ; illustrations by Lorie M. Gavulic; et al. (1999). Siegel GJ, Agranoff BW, Fisher SK, Albers RW, Uhler MD (ed.). Basic Neurochemistry (6 ed.). Lippincott Williams & Wilkins. ISBN 0-397-51820-X.
{{cite book}}
:|author=
has generic name (help); Explicit use of et al. in:|author=
(help)CS1 maint: multiple names: authors list (link) - ^ a b Rossier MF (2006). "T channels and steroid biosynthesis: in search of a link with mitochondria". Cell Calcium. 40 (2): 155–64. doi:10.1016/j.ceca.2006.04.020. PMID 16759697.
- ^ Brighton, Carl T.; Hunt, Robert M. (1974). "Mitochondrial calcium and its role in calcification". Clinical Orthopaedics and Related Research. 100 (100): 406–416. doi:10.1097/00003086-197405000-00057. PMID 4134194.
- ^ Brighton, Carl T. and Robert M. Hunt (1978): "The role of mitochondria in growth plate calcification as demonstrated in a rachitic model", Journal of Bone and Joint Surgery, 60-A: 630–639.
- ^ Pizzo P, Pozzan T (October 2007). "Mitochondria–endoplasmic reticulum choreography: structure and signaling dynamics". Trends Cell Bio. 17 (10): 511–517. doi:10.1016/j.tcb.2007.07.011. PMID 17851078.
- ^ a b Miller RJ (1998). "Mitochondria – the kraken wakes!". Trends in Neurosci. 21 (3): 95–97. doi:10.1016/S0166-2236(97)01206-X.
- ^ Schwarzlander M, Logan DC, Johnston IG, Jones NS, Meyer AJ, Fricker MD, Sweetlove LJ (2012). "Pulsing of Membrane Potential in Individual Mitochondria: A Stress-Induced Mechanism to Regulate Respiratory Bioenergetics in Arabidopsis". Plant Cell. 24 (3): 1188–201. doi:10.1105/tpc.112.096438. PMC 3336130. PMID 22395486.
{{cite journal}}
: CS1 maint: multiple names: authors list (link) - ^ Ivannikov, M.; et al. (2013). "Mitochondrial Free Ca2+ Levels and Their Effects on Energy Metabolism in Drosophila Motor Nerve Terminals". Biophys. J. 104 (11): 2353–2361. doi:10.1016/j.bpj.2013.03.064. PMC 3672877. PMID 23746507.
{{cite journal}}
: Explicit use of et al. in:|author=
(help) - ^ Li X, Fang P, Mai J; et al. (February 2013). "Targeting mitochondrial reactive oxygen species as novel therapy for inflammatory diseases and cancers". J Hematol Oncol. 6 (19): 19. doi:10.1186/1756-8722-6-19. PMC 3599349. PMID 23442817.
{{cite journal}}
: Explicit use of et al. in:|author=
(help)CS1 maint: multiple names: authors list (link) CS1 maint: unflagged free DOI (link) - ^ Green DR (September 1998). "Apoptotic pathways: the roads to ruin". Cell. 94 (6): 695–8. doi:10.1016/S0092-8674(00)81728-6. PMID 9753316.
- ^ Hajnóczky G, Csordás G, Das S, Garcia-Perez C, Saotome M, Sinha Roy S, Yi M (2006). "Mitochondrial calcium signalling and cell death: approaches for assessing the role of mitochondrial Ca2+ uptake in apoptosis". Cell Calcium. 40 (5–6): 553–60. doi:10.1016/j.ceca.2006.08.016. PMC 2692319. PMID 17074387.
{{cite journal}}
: CS1 maint: multiple names: authors list (link) - ^ McBride HM, Neuspiel M, Wasiak S (July 2006). "Mitochondria: more than just a powerhouse". Curr Biol. 16 (14): R551–60. doi:10.1016/j.cub.2006.06.054. PMID 16860735.
{{cite journal}}
: CS1 maint: multiple names: authors list (link) - ^ Oh-hama T (August 1997). "Evolutionary consideration on 5-aminolevulinate synthase in nature". Orig Life Evol Biosph. 27 (4): 405–12. doi:10.1023/A:1006583601341. PMID 9249985.
- ^ Klinge, Carolyn (2008). "Estrogenic Control of Mitochondrial Function and Biogenesis". J Cell Biochem. 105 (6): 1342–1351. doi:10.1002/jcb.21936.
- ^ Álvarez-Delgado, Carolina (2010). "Different expression of alpha and beta mitochondrial estrogen receptors in the aging rat brain: interaction with respiratory complex V.". Experimental Gerontology. 45 (7–8): 580–585. doi:10.1016/j.exger.2010.01.015.
- ^ Pavón, Natalia (2012). "Sexual hormones: effects on cardiac and mitochondrial activity after ischemia-reperfusion in adult rats. Gender difference". J Steroid Biochem Mol Biol. 132 (1–2): 135–146. doi:10.1016/j.jsbmb.2012.05.003.
- ^ Weinberg, Frank; Chandel, Navdeep S. (2009). "Mitochondrial Metabolism and Cancer". Annals of the New York Academy of Sciences. 1177 (1): 66–73. doi:10.1111/j.1749-6632.2009.05039.x.
- ^ a b Moreno-Sánchez, Rafael; Rodríguez-Enríquez, Sara; Marín-Hernández, Alvaro; Saavedra, Emma (2007). "Energy metabolism in tumor cells". FEBS Journal. 274 (6): 1393–1418. doi:10.1111/j.1742-4658.2007.05686.x. PMID 17302740.
- ^ Pedersen, Peter L. (1994). "ATP Synthase: The machine that makes ATP". Current Biology. 4 (12): 1138–1141. doi:10.1016/S0960-9822(00)00257-8. PMID 7704582.
- ^ Pattappa, Girish; Heywood, Hannah K.; de Bruijn, Joost D.; Lee, David A. (2011). "The metabolism of human mesenchymal stem cells during proliferation and differentiation". Journal of Cellular Physiology. 226 (10): 2562–2570. doi:10.1002/jcp.22605. PMID 21792913.
- ^ Agarwal, Bhawana (2011). "A role for anions in ATP synthesis and its molecular mechanistic interpretation". Journal of Bioenergetics and Biomembranes. 43 (3): 299–310. doi:10.1007/s10863-011-9358-3. PMID 21647635.
- ^ a b c Sweet, S.; Singh, G. (1999). "Changes in mitochondrial mass, membrane potential, and cellular adenosine triphosphate content during the cell cycle of human leukemic (HL-60) cells". Journal of Cellular Physiology. 180 (1): 91–96. doi:10.1002/(SICI)1097-4652(199907)180:1<91::AID-JCP10>3.0.CO;2-6. PMID 10362021.
- ^ McBride, Heidi M.; Neuspiel, Margaret; Wasiak, Sylwia (2006). "Mitochondria: More Than Just a Powerhouse". Current Biology. 16 (14): R551 – R560. doi:10.1016/j.cub.2006.06.054. PMID 16860735.
- ^ William F. Martin and Miklós Müller "Origin of mitochondria and hydrogenosomes", Springer Verlag, Heidelberg 2007.
- ^ Emelyanov VV (2003). "Mitochondrial connection to the origin of the eukaryotic cell". Eu J Biochem. 270 (8): 1599–1618. doi:10.1046/j.1432-1033.2003.03499.x. PMID 12694174.
- ^ Gray MW, Burger G, Lang BF (March 1999). "Mitochondrial evolution". Science. 283 (5407): 1476–81. doi:10.1126/science.283.5407.1476. PMID 10066161.
{{cite journal}}
: CS1 maint: multiple names: authors list (link) - ^ J. Cameron Thrash, Alex Boyd, Megan J. Huggett, Jana Grote, Paul Carini, Ryan J. Yoder, Barbara Robbertse, Joseph W. Spatafora, Michael S. Rappé, Stephen J. Giovannoni (June 2011). "Phylogenomic evidence for a common ancestor of mitochondria and the SAR11 clade" (PDF). Scientific Reports. 1. doi:10.1038/srep00013.
{{cite journal}}
: CS1 maint: multiple names: authors list (link) - ^ Ferla MP, Thrash JC, Giovannoni SJ, Patrick WM (2013). "New rRNA gene-based phylogenies of the Alphaproteobacteria provide perspective on major groups, mitochondrial ancestry and phylogenetic instability". PLOS ONE. 8 (12): e83383. Bibcode:2013PLoSO...883383F. doi:10.1371/journal.pone.0083383. PMC 3859672. PMID 24349502.
- ^ O'Brien TW (September 2003). "Properties of human mitochondrial ribosomes". IUBMB Life. 55 (9): 505–13. doi:10.1080/15216540310001626610. PMID 14658756.
- ^ Lynn Sagan (1967). "On the origin of mitosing cells". J Theor Biol. 14 (3): 255–274. doi:10.1016/0022-5193(67)90079-3. PMID 11541392.
- ^ Emelyanov VV (2001). "Rickettsiaceae, rickettsia-like endosymbionts, and the origin of mitochondria". Biosci. Rep. 21 (1): 1–17. doi:10.1023/A:1010409415723. PMID 11508688.
- ^ Feng D-F, Cho G, Doolittle RF (1997). "Determining divergence times with a protein clock: Update and reevaluation". Proc. Natl. Acad. Sci. 94 (24): 13028–13033. doi:10.1073/pnas.94.24.13028. PMC 24257. PMID 9371794.
{{cite journal}}
: CS1 maint: multiple names: authors list (link) - ^ Cavalier-Smith T (1991). "Archamoebae: the ancestral eukaryotes?". Biosystems. 25 (1–2): 25–38. doi:10.1016/0303-2647(91)90010-I. PMID 1854912.
- ^ a b c Chan DC (2006-06-30). "Mitochondria: Dynamic Organelles in Disease, Aging, and Development". Cell. 125 (7): 1241–1252. doi:10.1016/j.cell.2006.06.010. PMID 16814712.
- ^ a b Wiesner RJ, Ruegg JC, Morano I (1992). "Counting target molecules by exponential polymerase chain reaction, copy number of mitochondrial DNA in rat tissues". Biochim Biophys Acta. 183 (2): 553–559. doi:10.1016/0006-291X(92)90517-O. PMID 1550563.
{{cite journal}}
: CS1 maint: multiple names: authors list (link) Cite error: The named reference "Wiesner" was defined multiple times with different content (see the help page). - ^ Fukuhara H, Sor F, Drissi R, Dinouël N, Miyakawa I, Rousset, and Viola AM (1993). "Linear mitochondrial DNAs of yeasts: frequency of occurrence and general features". Mol Cell Biol. 13 (4): 2309–2314. PMC 359551. PMID 8455612.
{{cite journal}}
: CS1 maint: multiple names: authors list (link) - ^ Bernardi G (1978). "Intervening sequences in the mitochondrial genome". Nature. 276 (5688): 558–559. doi:10.1038/276558a0. PMID 214710.
- ^ Hebbar SK, Belcher SM, Perlman PS (April 1992). "A maturase-encoding group IIA intron of yeast mitochondria self-splices in vitro". Nucleic Acids Research. 20 (7): 1747–54. doi:10.1093/nar/20.7.1747. PMC 312266. PMID 1579468.
{{cite journal}}
: CS1 maint: multiple names: authors list (link) - ^ Gray MW, Lang BF, Cedergren R, Golding GB, Lemieux C, Sankoff D; et al. (1998). "Genome structure and gene content in protist mitochondrial DNAs". Nucleic Acids Research. 26 (4): 865–878. doi:10.1093/nar/26.4.865. PMC 147373. PMID 9461442.
{{cite journal}}
: Explicit use of et al. in:|author=
(help)CS1 maint: multiple names: authors list (link) - ^ Gray MW, Lang BF, Burger G (2004). "Mitochondria of protists". Ann Rev of Genetics. 38: 477–524. doi:10.1146/annurev.genet.37.110801.142526. PMID 15568984.
{{cite journal}}
: CS1 maint: multiple names: authors list (link) - ^ Telonis AG.; et al. (2014). "Nuclear and Mitochondrial tRNA-lookalikes in the Human Genome". Frontiers in Genetics. 5: 00344. doi:10.3389/fgene.2014.00344.
{{cite journal}}
:|first2=
missing|last2=
(help);|first3=
missing|last3=
(help);|first4=
missing|last4=
(help); Explicit use of et al. in:|author=
(help); Unknown parameter|author-separator=
ignored (help)CS1 maint: unflagged free DOI (link) - ^ Shao R, Kirkness EF, Barker SC (March 2009). "The single mitochondrial chromosome typical of animals has evolved into 18 minichromosomes in the human body louse, Pediculus humanus". Genome Res. 19 (5): 904–12. doi:10.1101/gr.083188.108. PMC 2675979. PMID 19336451.
{{cite journal}}
: CS1 maint: multiple names: authors list (link) - ^ Crick, F. H. C. and Orgel, L. E. (1973) "Directed panspermia." Icarus 19:341–346. p. 344: "It is a little surprising that organisms with somewhat different codes do not coexist." (Further discussion at [1] )
- ^ Barrell BG, Bankier AT, Drouin J (1979). "A different genetic code in human mitochondria". Nature. 282 (5735): 189–194. doi:10.1038/282189a0. PMID 226894.
{{cite journal}}
: CS1 maint: multiple names: authors list (link) - ^ NCBI "Mitochondrial Genetic Code in Taxonomy Tree"
- ^ NCBI: "The Genetic Codes", Compiled by Andrzej (Anjay) Elzanowski and Jim Ostell
- ^ Jukes TH, Osawa S (1990-12-01). "The genetic code in mitochondria and chloroplasts". Experientia. 46 (11–12): 1117–26. doi:10.1007/BF01936921. PMID 2253709.
- ^ Hiesel R, Wissinger B, Schuster W, Brennicke A (2006). "RNA editing in plant mitochondria". Science. 246 (4937): 1632–4. doi:10.1126/science.2480644. PMID 2480644.
{{cite journal}}
: CS1 maint: multiple names: authors list (link) - ^ Abascal F, Posada D, Knight RD, Zardoya R (2006). "Parallel Evolution of the Genetic Code in Arthropod Mitochondrial Genomes". PLOS Biology. 4 (5): 0711–0718. doi:10.1371/journal.pbio.0040127. PMC 1440934. PMID 16620150.
{{cite journal}}
: CS1 maint: multiple names: authors list (link) CS1 maint: unflagged free DOI (link) - ^ a b Henriquez FL, Richards TA, Roberts F, McLeod R, Roberts CW (February 2005). "The unusual mitochondrial compartment of Cryptosporidium parvum". Trends Parasitol. 21 (2): 68–74. doi:10.1016/j.pt.2004.11.010. PMID 15664529.
{{cite journal}}
: CS1 maint: multiple names: authors list (link) - ^ Pfeiffer, Ronald F. (2012). Parkinson's Disease. CRC Press. p. 583.
- ^ Seo AY, Joseph AM, Dutta D, Hwang JC, Aris JP, Leeuwenburgh C (August 2010). "New insights into the role of mitochondria in aging: mitochondrial dynamics and more". J. Cell. Sci. 123 (15): 2533–42. doi:10.1242/jcs.070490. PMC 2912461. PMID 20940129.
{{cite journal}}
: CS1 maint: multiple names: authors list (link) - ^ Hu GB (May 28, 2014). "Whole Cell Cryo-eletron tomography suggest mitochondria divide by budding". Microsc and microanal: 1–8. doi:10.1017/S1431927614001317.
- ^ Kimball, J.W. (2006) "Sexual Reproduction in Humans: Copulation and Fertilization," Kimball's Biology Pages (based on Biology, 6th ed., 1996)
- ^ Sutovsky, P.; et al. (1999). "Ubiquitin tag for sperm mitochondria". Nature. 402 (6760): 371–372. doi:10.1038/46466. PMID 10586873.
{{cite journal}}
: Explicit use of et al. in:|author=
(help) Discussed in Science News. - ^ Mogensen HL (1996). "The Hows and Whys of Cytoplasmic Inheritance in Seed Plants". American Journal of Botany. 83 (3): 383–404. doi:10.2307/2446172. JSTOR 2446172.
- ^ Zouros E (December 2000). "The exceptional mitochondrial DNA system of the mussel family Mytilidae". Genes Genet. Syst. 75 (6): 313–8. doi:10.1266/ggs.75.313. PMID 11280005. [dead link ]
- ^ Sutherland B, Stewart D, Kenchington ER, Zouros E (1 January 1998). "The fate of paternal mitochondrial DNA in developing female mussels, Mytilus edulis: implications for the mechanism of doubly uniparental inheritance of mitochondrial DNA". Genetics. 148 (1): 341–7. PMC 1459795. PMID 9475744.
{{cite journal}}
: CS1 maint: multiple names: authors list (link) - ^ Male and Female Mitochondrial DNA Lineages in the Blue Mussel (Mytilus edulis) Species Group by Donald T. Stewart, Carlos Saavedra, Rebecca R. Stanwood, Amy 0. Ball, and Eleftherios Zouros
- ^ Johns, D. R. (2003). "Paternal transmission of mitochondrial DNA is (fortunately) rare". Annals of Neurology. 54 (4): 422–4. doi:10.1002/ana.10771. PMID 14520651.
- ^ Fruit flies offer DNA clue to why women live longer
- ^ Thyagarajan B, Padua RA, Campbell C (1996). "Mammalian mitochondria possess homologous DNA recombination activity". J. Biol. Chem. 271 (44): 27536–27543. doi:10.1074/jbc.271.44.27536. PMID 8910339.
{{cite journal}}
: CS1 maint: multiple names: authors list (link) CS1 maint: unflagged free DOI (link) - ^ Lunt DB, Hyman BC (15 May 1997). "Animal mitochondrial DNA recombination". Nature. 387 (6630): 247. doi:10.1038/387247a0. PMID 9153388.
- ^ Eyre-Walker A, Smith NH, Maynard Smith J (1999-03-07). "How clonal are human mitochondria?". Proceedings of the Royal Society B. 266 (1418): 477–483. doi:10.1098/rspb.1999.0662. PMC 1689787. PMID 10189711.
{{cite journal}}
: CS1 maint: multiple names: authors list (link) - ^ Awadalla P, Eyre-Walker A, Maynard Smith J (24 December 1999). "Linkage Disequilibrium and Recombination in Hominid Mitochondrial DNA". Science. 286 (5449): 2524–2525. doi:10.1126/science.286.5449.2524. PMID 10617471.
{{cite journal}}
: CS1 maint: multiple names: authors list (link) - ^ Castro JA, Picornell A, Ramon M (1998). "Mitochondrial DNA: a tool for populational genetics studies". Int Microbiol. 1 (4): 327–32. PMID 10943382.
{{cite journal}}
: CS1 maint: multiple names: authors list (link) - ^ Cann RL, Stoneking M, Wilson AC (January 1987). "Mitochondrial DNA and human evolution". Nature. 325 (6099): 31–36. doi:10.1038/325031a0. PMID 3025745.
{{cite journal}}
: CS1 maint: multiple names: authors list (link) - ^ Torroni A, Achilli A, Macaulay V, Richards M, Bandelt HJ (2006). "Harvesting the fruit of the human mtDNA tree". Trends Genet. 22 (6): 339–45. doi:10.1016/j.tig.2006.04.001. PMID 16678300.
{{cite journal}}
: CS1 maint: multiple names: authors list (link) - ^ a b Garrigan D, Hammer MF (2006). "Reconstructing human origins in the genomic era". Nature Reviews Genetics. 7 (9): 669–80. doi:10.1038/nrg1941. PMID 16921345.
- ^ Krings M, Stone A, Schmitz RW, Krainitzki H, Stoneking M, Pääbo S (1997). "Neandertal DNA sequences and the origin of modern humans". Cell. 90 (1): 19–30. doi:10.1016/S0092-8674(00)80310-4. PMID 9230299.
{{cite journal}}
: CS1 maint: multiple names: authors list (link) - ^ Harding RM, Fullerton SM, Griffiths RC, Bond J, Cox MJ, Schneider JA, Moulin DS, Clegg JB (April 1997). "Archaic African and Asian lineages in the genetic ancestry of modern humans". American Journal of Human Genetics. 60 (4): 772–89. PMC 1712470. PMID 9106523.
{{cite journal}}
: CS1 maint: multiple names: authors list (link) - ^ a b c Zeviani M, Di Donato S (2004). "Mitochondrial disorders". Brain. 127 (Pt 10): 2153–2172. doi:10.1093/brain/awh259. PMID 15358637.
- ^ Taylor RW, Turnbull DM (2005). "MITOCHONDRIAL DNA MUTATIONS IN HUMAN DISEASE". Nature Reviews Genetics. 6 (5): 389–402. doi:10.1038/nrg1606. PMC 1762815. PMID 15861210.
- ^ Chinnery PF, Schon EA (2003). "Mitochondria". J. Neurol. Neurosurg. Psychiatr. 74 (9): 1188–99. doi:10.1136/jnnp.74.9.1188. PMC 1738655. PMID 12933917.
- ^ Sherer TB, Betarbet R, Greenamyre JT (2002). "Environment, mitochondria, and Parkinson's disease". The Neuroscientist. 8 (3): 192–7. doi:10.1177/1073858402008003004. PMID 12061498.
{{cite journal}}
: CS1 maint: multiple names: authors list (link) - ^ Gomez C, Bandez MJ, Navarro A (2007). "Pesticides and impairment of mitochondrial function in relation with the parkinsonian syndrome". Front. Biosci. 12: 1079–93. doi:10.2741/2128. PMID 17127363.
{{cite journal}}
: CS1 maint: multiple names: authors list (link) - ^ Lim YA; Rhein, Virginie; Baysang, Ginette; Meier, Fides; Poljak, Anne; j. Raftery, Mark; Guilhaus, Michael; Ittner, Lars M.; Eckert, Anne; et al. (2010). "Abeta and human amylin share a common toxicity pathway via mitochondrial dysfunction". Proteomics. 10 (8): 1621–33. doi:10.1002/pmic.200900651. PMID 20186753.
{{cite journal}}
: Explicit use of et al. in:|author=
(help); Unknown parameter|displayauthors=
ignored (|display-authors=
suggested) (help) - ^ Schapira AH (2006). "Mitochondrial disease". Lancet. 368 (9529): 70–82. doi:10.1016/S0140-6736(06)68970-8. PMID 16815381.
- ^ Pieczenik SR, Neustadt J (2007). "Mitochondrial dysfunction and molecular pathways of disease". Exp. Mol. Pathol. 83 (1): 84–92. doi:10.1016/j.yexmp.2006.09.008. PMID 17239370.
- ^ Bugger H, Abel ED (2010). "Mitochondria in the diabetic heart". Cardiovascular Research. 88 (2): 229–240. doi:10.1093/cvr/cvq239. PMC 2952534. PMID 20639213.
- ^ Richter C, Park J, Ames BN (September 1988). "Normal oxidative damage to mitochondrial and nuclear DNA is extensive". PNAS. 85 (17): 6465–6467. doi:10.1073/pnas.85.17.6465. PMC 281993. PMID 3413108.
{{cite journal}}
: CS1 maint: multiple names: authors list (link) - ^ Harman D (1956). "Aging: a theory based on free radical and radiation chemistry". J. Gerontol. 11 (3): 298–300. doi:10.1093/geronj/11.3.298. PMID 13332224.
- ^ Harman, D (1972). "A biologic clock: the mitochondria?". Journal of the American Geriatrics Society. 20 (4): 145–147. PMID 5016631.
- ^ Soares et al, Correcting for Purifying Selection: An Improved Human Mitochondrial Molecular Clock Am J Hum Genet. 2009 June 12; 84(6) 740–759.
- ^ Michael W. Nachman and Susan L. Crowell Estimate of the Mutation Rate per Nucleotide in Humans Genetics, Vol. 156, 297–304, September 2000
- ^ "Mitochondria and Aging".
- ^ Boffoli D, Scacco SC, Vergari R, Solarino G, Santacroce G, Papa S (1994). "Decline with age of the respiratory chain activity in human skeletal muscle". Biochim. Biophys. Acta. 1226 (1): 73–82. doi:10.1016/0925-4439(94)90061-2. PMID 8155742.
{{cite journal}}
: CS1 maint: multiple names: authors list (link) - ^ de Grey, Aubrey (Fall 2004). "Mitochondrial Mutations in Mammalian Aging: An Over-Hasty About-Turn?" Rejuvenation Res. 7 (3) 171–4. .doi:10.1089/rej.2004.7.171 PMID 15588517.
- ^ Bender A, Krishnan KJ, Morris CM, Taylor GA, Reeve AK, Perry RH, Jaros E, Hersheson JS, Betts J, Klopstock T, Taylor RW, Turnbull DM (2006). "High levels of mitochondrial DNA deletions in substantia nigra neurons in aging and Parkinson disease". Nat Gen. 38 (5): 515–517. doi:10.1038/ng1769. PMID 16604074.
{{cite journal}}
: CS1 maint: multiple names: authors list (link) - ^ Trifunovic A, Hansson A, Wredenberg A, Rovio AT, Dufour E, Khvorostov I, Spelbrink JN, Wibom R, Jacobs HT, Larsson NG (2005). "Somatic mtDNA mutations cause aging phenotypes without affecting reactive oxygen species production". PNAS. 102 (50): 17993–8. doi:10.1073/pnas.0508886102. PMC 1312403. PMID 16332961.
{{cite journal}}
: CS1 maint: multiple names: authors list (link)
- General
This article incorporates public domain material from Science Primer. NCBI. Archived from the original on 2009-12-08.
External links
- Mitodb.com – The mitochondrial disease database.
- Mitochondria Atlas at University of Mainz
- Mitochondria Research Portal at mitochondrial.net
- Mitochondria: Architecture dictates function at cytochemistry.net
- Mitochondria links at University of Alabama
- MIP Mitochondrial Physiology Society
- 3D structures of proteins from inner mitochondrial membrane at University of Michigan
- 3D structures of proteins associated with outer mitochondrial membrane at University of Michigan
- Mitochondrial Protein Partnership at University of Wisconsin
- MitoMiner – A mitochondrial proteomics database at MRC Mitochondrial Biology Unit
- Mitochondrion – Cell Centered Database
- Mitochondrion Reconstructed by Electron Tomography at San Diego State University
- Video Clip of Rat-liver Mitochondrion from Cryo-electron Tomography